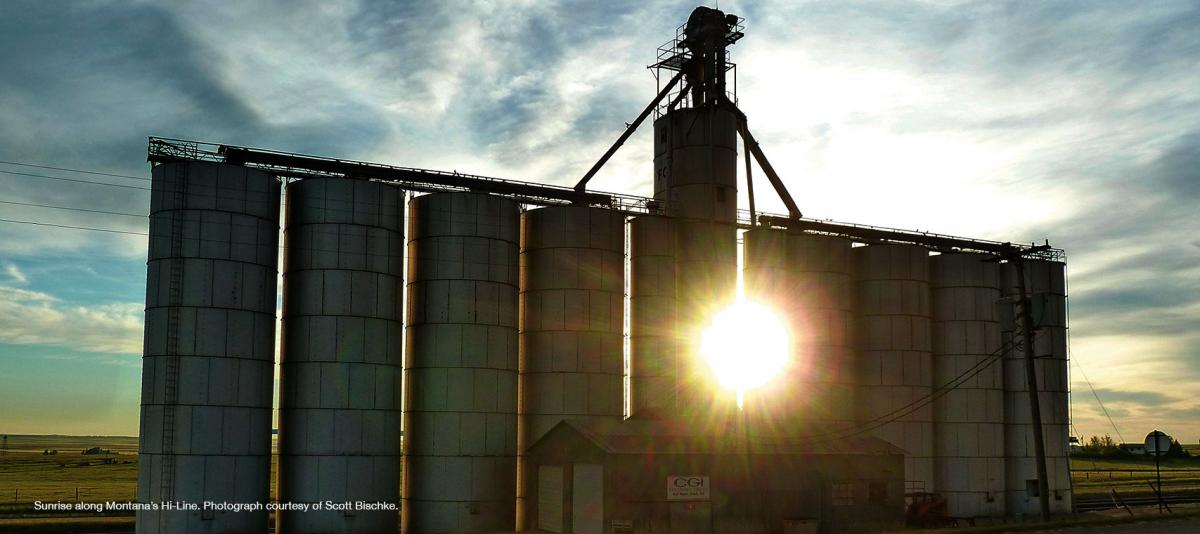
CHAPTER 5
AGRICULTURE AND CLIMATE CHANGE IN MONTANA
Bruce Maxwell, Becky Weed, Laura Ippolito, Anton Bekkerman, Madison Boone, Megan Mills-Novoa, David Weaver, Mary Burrows, and Laura Burkle
Montana agriculture has always faced variability and occasional extreme events. Wry commentary about the challenges of such variability might even be called a defining trait of rural culture in Montana. Characterizing the impacts of global climate change on Montana’s diverse and historically variable agriculture is not clear cut. In the Third National Climate Assessment Melillo et al. (2014) described the challenge, though for the country as a whole, as follows:
The cumulative effects of climate change will ultimately depend on changing global market conditions as well as responses to local climate stressors, including farmers adjusting planting patterns in response to altered crop yields and crop species, seed producers investing in drought-tolerant varieties, and nations restricting trade to protect food security. Adaptive actions in the areas of consumption, production, education, and research involve seizing opportunities to avoid economic damages and decline in food quality, minimize threats posed by climate stress, and in some cases increase profitability.
In other words, any effort at assessing climate impacts on agriculture faces multiple layers of uncertainty, including uncertainty that 1) accompanies all climate projections, 2) is specific to agricultural projections, and 3) is created by adaptive actions (human interventions) that can mask a direct climate impact signal.
Consequently, in the chapter that follows we emphasize and place higher confidence in projections that are consistent with current climate trends and supported by agricultural data. We must also acknowledge longer-term climate projections that may not yet be manifest as agricultural impacts. We first provide a summary of key climate projections relevant for Montana agriculture, followed by a brief overview of the uncertainties associated with identifying and predicting climate change effects. We next review the influence of climate change on Montana crops and livestock, and on the associated roles of pollinators, disease, pests, and weeds. We also report a number of human adaptations already underway that may increase resilience in the face of climate change. This combination of uncertain projections, local and global effects, and potential for human adaptation makes it difficult to attribute current, much less future, changes and trends in Montana agriculture solely to climate change. Accepting the reality of that uncertainty, we conclude the chapter by discussing future challenges for the agricultural sector related to climate change and the next steps for research and assessment.
KEY MESSAGESThere are multiple drivers of decision-making in agriculture. Climate change is only one of the drivers motivating agricultural innovation, but it will become more important as warming continues into the future. [high agreement, robust evidence] Every component of agriculture—from prices to plant pollinators and crop pests—exhibits complex relationships to climate, depending on the location, weather variability, and agricultural and economic practices and policies. Social and economic resilience to withstand and adapt to variable conditions has always been a hallmark of Montana farmers’ and livestock producers’ strategies for coping with climate variability. [high agreement, robust evidence] Projected temperature and precipitation increases may be favorable in the short term for some Montana crops and forage production, but the effects of warming will become increasingly disruptive as they accelerate beyond adaptation thresholds. More frost-free days and longer growing seasons will potentially enable greater crop diversity. However, more 90°F+ (32°C+) days will also 1) increase evapotranspiration and water demand for most crops; 2) limit grain development from pollination to seed (i.e., grain fill); and 3) elevate heat stress on livestock. [medium agreement, medium evidence] Decreasing mountain snowpack will continue to lead to decreased streamflow and less reliable irrigation capacity during the late growing season. Reduced irrigation capacity will have the greatest impact on hay, sugar beet, malt barley, market garden, and potato production across the state. [high agreement, robust evidence] Climate change affects global-price-determined commodity agriculture differently than it affects non-commodity agriculture. Commodity crops, such as small grains, are more directly driven by global markets and agricultural subsidies, whereas non-commodity crops tend to be more directly tied to local or specialized non-local markets and local micro-climates. [high agreement, medium evidence] Diversified cropping systems, including rotation with pulse crops and innovations in tillage and cover-cropping, along with other measures to improve soil health, will continue to allow adaptation to climate change. [medium agreement, low evidence] Models predict native plains vegetation will increase but livestock forage quality will decrease. [medium agreement, low evidence] Increases in temperature will allow winter annual weeds, such as cheatgrass, to increase in distribution and frequency in winter wheat cropland and rangeland. Their spread will result in decreased crop yields and forage productivity, as well as increased rangeland wildfire frequency. [high agreement, medium evidence] Projected increases in winter temperature and spring precipitation are likely to increase current crop diseases and pests. For example, increased planting of winter wheat will be accompanied by increased crop pests, such as wheat stem sawfly, and the natural regulation of this pest by native parasitoids will likely decline. [medium agreement, medium evidence] |
||
BACKGROUND
Agriculture is a key industry in Montana, generating over $5.2 billion in 2014 through the sale of agricultural commodities (USDA-NASS 2015). Montana’s large agricultural industry consists of both crops and livestock, as summarized by revenue in Table 5-1.
Commodity | Value (US dollars) | National Rank | % US Total | Cropland Acres in MT Planted | Proportion of Total Cropland Acres in MT | Proportion of All Land in Farms and Ranches |
Durum Wheat | $125,969,000 | 2 | 25.1 | 435,000 | 2.55% | 0.73% |
Other Spring Wheat | $634,144,000 | 2 | 17.5 | 3,050,000 | 17.91% | 5.10% |
Winter Wheat | $538,182,000 | 2 | 6.7 | 2,500,000 | 14.68% | 4.18% |
Barley | $238,038,000 | 2 | 25.3 | 920,000 | 5.40% | 1.54% |
Fruits, vegetables, melons, and tree nuts | $192,814,000 | n/a | n/a | n/a | n/a | n/a |
Lentils | $40,151,000 | 1 | 52.3 | 130,000 | 0.76% | 0.22% |
Dry Edible Peas | $99,792,000 | 1 | 52.9 | 525,000 | 3.08% | 0.88% |
Austrian Winter Peas | not available | 2 | 31.1 | 12,000 | 0.07% | >0.02% |
Sugar beets | $49,250,000 | 5 | 4.6 | 45,100 | 0.26% | 0.08% |
Potatoes | $46,285,000 | 13 | 0.9 | 11,500 | 0.07% | 0.02% |
Corn (grain + silage) | $28,125,000 | 36 | 0.1 | 130,000 | 0.76% | 0.22% |
Oats | $3,643,000 | 17 | 1.6 | 45,000 | 0.26% | 0.08% |
All Hay | $668,427,000 | 8 | 3.9 | 2,730,000 | 16.03% | 4.57% |
Livestock | Gross Income(dollars) | National Rank | % US Total | Pasture and Range Acres in MT | Proportion of Total Pasture and Range Acres in MT | Proportion of All Land in Farms and Ranches |
All cattle and calves | $2,014,017,000 | 11 | 2.8 | n/a | n/a | n/a |
Hogs and pigs | $78,612,000 | 23 | 0.3 | n/a | n/a | n/a |
Dairy products | $65,560,000 | n/a | n/a | n/a | n/a | n/a |
All sheep | $50,525,000 | 8 | 4.1 | n/a | n/a | n/a |
Honey | $29,225,000 | 4 | 8 | n/a | n/a | n/a |
Eggs | $12,966,000 | 35 | 0.1 | n/a | n/a | n/a |
Chickens | $3,100,000 | 35 | 0.1 | n/a | n/a | n/a |
Agriculture plays a dominant role in the state’s land use and its people’s sense of place. Thus, even though more Montanans live in cities than on farms and ranches (USDA Census of Agriculture 2012), many of them think of Montana as an agricultural state, where the non-forested landscape is dominated by livestock and crop production.
Montana’s farm and ranchland is a mosaic of dryland and irrigated agriculture, commodity and specialty cropland, and native and planted rangeland, all set on a backdrop of public and private lands that represent a spectrum from cities to wildlands. The analysis in this chapter separates Montana into seven agricultural regions (USDA-NASS 2015), which correspond to the seven NOAA-defined, Montana climate divisions in Figure 2-3 (Climate chapter). The seven agricultural regions are characterized as follows:
- Northwestern and southwestern.—The mountain valleys of the northwestern and southwestern regions are dominated by hay and livestock production with a few isolated areas of small grains, seed potatoes, malt barley, and other rotational crops. In addition, this region has irrigated, small-scale market garden and orchard crops surrounding urban centers and Flathead Lake.
- Central.—The southern portions of the central region are dominated by livestock and hay production. A large part of the area is irrigated, with some isolated small-grain production.
- North central.—The Golden Triangle, known primarily for its wheat production, represents a large part of the north central region. The region is dominated by dryland, small-grain production (with alternate fallow years to store soil moisture), with some legume and oil seed rotational crops. Livestock agriculture is less important than in other regions of the state.
- South central.—The west half of the south central region is dominated by livestock and associated irrigated hay production. The east half (Yellowstone, Big Horn, and Treasure counties) is characterized by river valleys with irrigated crops and by dryland winter wheat production.
- Northeastern.—The northeastern region is dominated by dryland small-grain production, including spring wheat with more continuous cropping by rotation with legume and oil seed crops. Livestock agriculture is less important than in other regions of the state.
- Southeastern.—The southeastern region includes extensive rangeland with cattle production, dryland winter wheat, and some rotation with oil seed crops. Row crops, including sugar beets, dominate the river valleys, with corn and soybean production increasing.
Agricultural irrigation is generally most extensive in the southwestern quadrant of the state, but there are pockets of irrigation dependence throughout Montana that do not correlate strictly with the regional divisions. The Water chapter of this document addresses climate impacts to water supply issues and more extensive documentation of agricultural irrigation in Montana is available in the 2015 Montana State Water Plan (MT DNRC 2015). The DNRC Plan includes maps and assessments of hydrologic basins and irrigation infrastructure, including context of climate projections. We do not reproduce those data on a region-by-region basis, but we do discuss the relationship between irrigated crops and climate change.
SUMMARY OF KEY CLIMATE PROJECTIONS FOR MONTANA AGRICULTURE
As described in the Climate chapter of this assessment, average annual temperatures in Montana increased from 2.7°F (1.5°C) between 1950 and 2015, with even higher warming occurring in winter and spring (3.6°F [2.0°C] and 2.6°F [1.4°C], respectively). As a result, the annual growing season lengthened during roughly the same period by 12 days. Average annual precipitation for Montana, in contrast, did not change markedly between 1950 and 2015.
Climate model projections show a warmer Montana in the future, with mixed changes in precipitation, more extreme events, and mixed certainty on upcoming drought. As the basis for the chapter to follow, we provide summaries of the scaled-down global climate model projections for each of these climate variables below. More in-depth information can be found in the Climate chapter of this assessment.
- Temperature projections.—The state of Montana will continue to warm in all geographic locations, seasons, and under all modeled global emission scenarios, throughout the 21st century. By mid century and end-of-century, respectively, Montana temperatures are projected to increase by roughly 4.5-6.0°F (2.5-3.3°C) and 5.6-9.8°F (3.1-5.4°C), depending on emission scenarios. These projected temperature increases are larger than the average changes projected globally and nationally.
- Precipitation projections.—Across the state, precipitation (rain and snow) will increase in winter, spring, and fall. Precipitation is expected to decrease in summer. The largest increases are expected to occur during spring in the southern part of the state, with increases of 0.2-0.4 inches/month (0.5-1.0 cm/month) and 0.4 inches/month (1.0 cm/month) expected by mid and end-of-century, respectively, depending on emission scenarios. The largest decreases are expected to occur during summer in the central and southern parts of the state (0.2 inches/month [0.5 cm/month] by end-of-century under two emission scenarios).
- Extreme events.—Agricultural productivity is highly vulnerable to extreme weather events, such as flooding, blizzards, hailstorms, and drought (Melillo et al. 2014). Although it is not possible to predict the precise location, magnitude and timing of such events in the future, more extreme events, as part of increased climate variability, may impact agricultural systems over and above those impacts associated with gradual climate change (Harrison et al. 2016). For example, crop-damaging hail events have consistently occurred in south central Montana in July from 1901-1980, with a slight increase in frequency from 1960-1980 (Changnon 1984). Recent predictions of hail threat over North America indicate that southwest and eastern Montana will see a significant increase in severe hail days in spring and early summer in 2041-2070 compared to 1971-2000 (Brimelow et al. 2017). Potentially damaging hail events for agriculture in Montana are generally predicted to increase if one assumes that hail larger than 0.4 inches (1 cm) in diameter is likely to damage crops or livestock (Figure 5-1). Brimelow et al. (2017) used dynamically downscaled data (on a 31-mile [50-km] grid) from the North American Regional Climate Change Assessment Program as input for HAILCAST—a computationally efficient, one-dimensional cloud model linked to a time-dependent hail growth model with microphysics to simulate the growth and melting of hail from first principles. Hail has its greatest impact on barley and wheat once heads with grain are formed (currently, early June for winter wheat and late June or early July for spring wheat and barley). Hail measuring 0.4 inches (1 cm) is enough to significantly damage small grain crops (Sanchez et al. 1996). The predicted increase (ΔGE1in Figure 5-1) of days of small grain damaging hail could result in increased hail damage insurance premiums, which further challenges the economics of Montana crop production.
Projected Changes in Hail Events
Figure 5-1. Mean changes in hail (diameter ≥ 1.0 cm) event days per season from the present (1971–2000) to the future (2041–2070) for spring (March-May) (left) and summer (June-August) (right) based on multiple model simulations. Colored cells indicate mean changes for all model pairings that agree on the direction of change; cells with colored circles indicate mean changes for at least two model pairings (Brimelow et al. 2017). - Drought.—Drought is more difficult to predict under a future with increasing greenhouse gas emissions. Drought seasonality, duration, frequency, and intensity all strongly impact agriculture—for example, reducing levels of soil moisture to support crop growth—and the lack of predictability under climate change is problematic. For Montana, increasing temperatures will likely intensify drought when it occurs, but precipitation projections do not reveal increasing duration or frequency of drought. When drought is discussed in the remainder of this chapter, it is referring to agricultural drought as defined in the Drought sidebar of the Climate chapter.
SOURCES OF UNCERTAINTY
Uncertainty accompanies efforts to assess the impacts of climate change on agriculture, including uncertainty in climate modeling (e.g., Melillo et al. 2014), in crop growth modeling (e.g., Ruane et al. 2016), in predicting livestock production, and in economic projections. Similarly, agricultural responses, largely driven by economics, may vary widely in the face of both local and global climate change. Such responses—also called adaptive actions—include altered planting and harvest dates, altered tillage and cover-cropping to manage water and weeds, adaptive grazing management, price support programs and other government subsidies, creation of specialized marketing channels, changes in crop selection, and crop insurance programs.
Climate change can affect all sectors of the agricultural industry, although in different ways at different scales, both directly and indirectly (Figure 5-2). For example, in considering agricultural markets: a) commodity grain revenues are affected by worldwide commodity yields and prices, which in turn might be impacted by global climate change; b) agricultural products marketed to consumers through local outlets (e.g., at farmers markets) can be affected by Montana’s climate, and are less impacted by global price fluctuations; c) livestock revenues can be affected by climate through prices of input commodities or shifts in range availability or in markets; and d) sea level change may require relocation of port facilities that are critical to Montana grain exports and therefore could decrease price received or increase the cost of transportation, making grain farming less profitable and sustainable.
Interactions of Natural Systems and Human Interventions |
![]() |
Figure 5-2. Interactions of natural systems and human interventions guarantee that climate change effects on agriculture, and vice versa, will be neither simple nor trivial. |
Crop growth models are simulations that help estimate crop yield based on multiple projected growing conditions. While such models have not been run explicitly for Montana, they have been applied to other locations and can provide insights—though sometimes with conflicting results—for considering impact on Montana wheat. Highlights from those models include:
- In a European study on wheat, the ensemble crop model indicated that average yields would decline 3 to 7% per 1.8°F (1°C) increase in temperature (Pirttioja et al. 2015).
- Under projected climate conditions somewhat similar to Montana in Australia, dryland wheat yield loss was predicted to range from 24-94% by the 2060s depending on the site and the regional climate projection (Kouadio et al. 2015).
- Thirty different models for predicting global wheat grain yield indicated high levels of uncertainty when simulating crop responses to increasing temperatures. The research indicated that the median wheat grain production would fall 6% per 1.8°F (1°C) increase in temperature, plus become more variable over space and time (Asseng et al. 2015).
- Ruane et al. (2016) compared 27 wheat model global yield responses to interannual climate variability and concluded that there is only a weak relationship (R2 ≤ 0.24) between the model sensitivities to interannual temperature variability and the crops’ actual response to long-term warming. This finding suggests that the models do not capture all the significant processes that affect wheat yield. Thus, the use of the physiologically-based crop-growth models to project climate responses may be highly uncertain without further refinements (Macadam et al. 2016).
- Results from a study of the Canadian prairie, the northernmost portion of the Great Plains of North America and adjacent to Montana grasslands, provide stark contrast to those described in the previous example (Smith et al. 2013). Researchers ran growth models using historical weather (1961–1990) and future climate scenarios (2040–2069; using IPCC Special Report on Emissions Scenarios). This study predicted that if no cultivar changes occurred, spring and winter wheat yields would increase by 37% and 70%, respectively. The indication is that northern regions are likely to see strong shifts toward increased agricultural productivity under climate change.
Results of these modeling studies, particularly from those from regions similar to Montana, are useful only with important caveats. For example, differences in temperature between Canada and Montana, and uncertainties in precipitation projections, influence crop model projections and call into question the ability to extrapolate the findings across major subregions within the Great Plains.
Even while the spectrum of modeling approaches used in these studies yield insights about variables that influence crop growth, it is apparent that absolute projections are not possible. Uncertainty exists for crop models, just as for climate models, and this must be acknowledged. Stakeholders of Montana agriculture may find the cumulative uncertainty of inexact crop models built on inexact climate models frustrating, but it is as important to understand the sources of uncertainty as it is to realize that temperatures are rising. Still, with temperatures rising and a strong need to understand the consequences for Montana agriculture, models provide our best tool for looking ahead. Models provide producers with a range of plausible scenarios to consider in designing adaptation strategies.
CLIMATE CHANGE EFFECTS ON COMMODITY CROPS IN MONTANA
Some of the crop production trends expected to accompany increasing temperatures are already apparent in statewide agricultural statistics compilations. The documented shifts may be attributable to climate change, but other factors may also contribute, in whole or in part. Due to the complex interplay of direct and indirect factors illustrated in Figure 5-2, the literature contains little documentation of climate change alone as being responsible for observed changes in Montana crop production.
As noted in the Key Climate Projections for Montana section (above), precipitation is projected to increase in some regions, and in some seasons, but not in others. This means that precipitation projections cannot be applied uniformly across the state, whereas temperature trends are more consistent statewide. Therefore, in this chapter we discuss observed and expected patterns of change for each of the major types of agricultural production rather than applying the climate trends equivalently across agricultural topics.
Shifting ratios of spring and winter wheat
Wheat is the number one commodity crop grown in Montana (Table 5-1). It has a production value of $939 million (USDA-NASS 2015), so changes in its acreage and distribution have significant implications for Montana’s economy and agricultural practices.
A shift from spring wheat towards winter wheat production is expected, due largely to warmer winter temperatures that facilitate greater winter wheat survival, and warmer summer temperatures that impair spring wheat production by inhibiting seed formation, germination, and early growth (Lanning et al. 2010). The increasing proportion of Montana winter wheat since 2000 (Figure 5-3) may be attributable to climate change in particular because of a) more consistent autumn precipitation, b) warmer winters, and c) heat damage to later maturing spring wheat. This shift to winter wheat is expected to increase in the future as winter temperatures and summer days above 90°F (32°C) increase.
|
![]() |
Figure 5-3. The proportion of total wheat acres planted each year in Montana as winter wheat (USDA-NASS 2015). |
However, while such a shift has already been documented in some places (e.g., Prato and Qui 2013), many factors—including local or global price—can complicate crop preference shifts. Figure 5-3 shows two historical trends for winter wheat production that are most likely not attributable to climate change:
- The increase in winter wheat from 1925-1970 resulted from improved cultivars bred for Montana conditions, not climate change.
- The relative decline in winter wheat acreage from 1987-2000 was probably driven more by the Conservation Reserve Program (CRP) than by direct climate effects. The Conservation Reserve Program gave favorable rates to Montana producers, leading many to remove acres from wheat production and move them into CRP.
Importantly, the factors driving a farmer’s choice to switch, for example, from wheat to a high-value rotational crop (e.g., lentils, corn) may change from year to year. Along with projected market price, farmers must balance these choices against myriad other considerations, including other crops and livestock on their land, government programs (e.g., CRP), labor scheduling, crop insurance, crop rotations, and family traditions.
Still, if current upward temperature trends continue or even accelerate, it is likely that the shift from spring wheat to winter wheat will continue. However, further analysis of crop selections and commodity pricing inside and outside Montana show, as discussed below, that projections regarding wheat cannot function in isolation.
Increased corn production
Corn acreage, and to a lesser degree soybean acreage, has increased across much of Montana since 1990, particularly in eastern Montana (Figure 5-4) (USDA-NASS 2015). Farmers can now grow corn in many areas where length of growing season, as well as spring and early fall temperatures, were formerly prohibitive. But in addition to the longer growing seasons, which may be driven by climate change (see Climate chapter), this improved feasibility of corn production in Montana is due in part to new, shorter-season corn varieties.
|
![]() |
Figure 5-4. Acres of corn planted each year in Montana, including that grown for silage (USDA-NASS 2015). |
Along with increasing temperatures and length of growing season, however, a combination of economic factors has favored the choice to plant corn in recent years:
- Profitability.—Corn has historically been more consistently profitable than many other crops, as indicated by comparisons of net return on labor and management in North Dakota (Aakre 2013; Newton and Kuethe 2015). This profit stability is due in large part to the major global market share that the US holds in corn production (40-45%). Therefore, US corn prices are not as sensitive to global conditions as wheat prices are. The US produces only about 7-9% of global wheat, causing wheat prices to be more dependent on what happens globally. Wheat price stability is also affected by discounts based on protein content, test weight, and weed seed dockage, whereas corn price is not so substantially affected by quality.
- Flexibility.—Farmers can harvest corn as silage for livestock feed, if necessary, even if the crop does not reach maturity. Thus, some Montana farmers are experimenting with corn acreage, even where there is still risk of early frosts terminating growth before maturity. Some Montana farmers may also be attracted to the option of using genetically modified, glyphosate-resistant corn to ease weed management, following a trend that has dominated agriculture in the midwestern US.
Whether this increasing corn acreage is being encouraged by warmer growing conditions caused by climate change, economic factors, or both, this expansion raises broader concerns about how crop selections will be made in a changing climate. Corn is an extremely water- and fossil-fuel-intensive crop typically grown as animal feed or biofuel, not as food for people. This allocation of resources is already the subject of debate with respect to midwestern corn, and there may be more pressure to adopt corn production in Montana with warming and increased precipitation as much of the continent becomes more arid. However, disease considerations may also play into crop selection trends, as wheat and barley growers are already raising concerns about corn as a disease carrier (see section on crop diseases).
Price volatility and the cost of uncertainty in commodity markets
The likelihood of increasingly volatile weather, both locally and globally, due to climate change will increase uncertainty in both local and global markets. In commodity markets, that uncertainty has a cost that relates not only to weather, but also to myriad choices involving forward contracting, futures marketing, crop selection, and crop quality.
Any agricultural decision has multiple drivers (Figure 5-5), but the discussion in this section applies specifically to the major commodity markets of Montana, where small grains, especially wheat, are dominant and pulses and corn are subsidiary. The most direct determinants of cropping decisions (i.e., crop selection) include input costs, pest conditions, government policies, and year-to-year price expectations.
|
![]() |
Figure 5-5. Factors that drive agricultural decisions in Montana. The size of bubble and arrows qualitatively represents the relative importance of each factor’s influence on agricultural production decisions. |
Climate interacts with all of these other variables shown in Figure 5-5, both directly and indirectly. Increasing uncertainty due to complex interactions, whether through volatility or new and hard-to-predict temperature and moisture trends, can disrupt agricultural decision-making and will probably become an even more important direct agriculture decision-driver in the years ahead. Climate change can impact the economics of Montana’s commodity crop industry in three principal ways:
- Agricultural producers and grain handlers are likely to be exposed to greater market uncertainty because of climate change. That uncertainty, in turn, may be incorporated into an operation’s cost structure, potentially leading to higher costs for both producers and marketers of the commodity.
- Climate change, and specifically rising temperatures, can alter Montana’s ability to consistently produce high-quality, higher-protein spring wheat, a key market differentiator for the state. Such a change could reduce Montana’s competitive advantage in global wheat markets and, as a result, reduce the economic returns from our state’s agricultural sector.
- Changes to Montana’s climate will likely alter the traditional selection of crops produced in the state. This change would alter Montana’s role on the US and global crop marketing landscape, although whether these impacts will be positive or negative is uncertain.
The preceding sections of this document address climate impacts on wheat quality and crop selection (shifts to winter wheat or corn), but the economics of price uncertainty is also a consequence of climate uncertainty. The accompanying Basis and Climate Change sidebar introduces the concept of basis, an economist’s tool for evaluating local and global influences on commodity prices; a more detailed analysis is provided in the appendices to this document.25 For the overall purposes of this climate assessment there is a bottom line: if errors exist in basis forecast, the costs of forward contracting will increase beyond the “usual” risk premiums. Such errors could potentially result from economic models that fail to incorporate climate complexities, or do so poorly. In laymen’s terms, price volatility builds upon the climate uncertainties of farming, and vice versa.
|
||
Basis and Climate Change Basis is an agricultural economists’ fundamental tool for understanding how markets incorporate new information into prices, including issues associated with climate change effects.
Basis (in $/bushel) = futures market price - local price where futures market price reflects global conditions local price reflects Montana production conditions
Basis can be used to assess differential impacts of climate change on local and global agricultural markets because it helps characterize how Montana-specific crop prices (reflective of local production conditions) are related to prices in futures markets (reflective of global conditions). If climate change leads to basis becoming more negative (or less positive) relative to historical averages—that is, the local price decreases relative to the futures price—this would imply that the impacts of climate change likely affected local prices more adversely than global prices. Conversely, rising basis would mean that local production and marketing conditions were less adversely impacted by climate change relative to global conditions. Therefore, basis enables the analysis of wheat economics at a local level while accounting for global market conditions. |
||
Pulse crops
Agricultural land planted with pulse crops (e.g., lentils, chickpeas, dry peas) has increased over the last 10-15 yr in Montana, with the northeastern region of the state leading the trend (Miller et al. 2002; Zentner et al. 2002; Cutforth et al. 2007; Burgess et al. 2012). Pulse crops provide multiple benefits to Montana farmers, benefits where management for climate change and management for other dimensions of farm health have the potential to converge. For example:
- Pulse crops enable farmers to diversify their production, thereby providing resilience in the face of climate change.—Diversification a) helps farmers cope with increasing climate-related variability in temperature and precipitation, and b) provides some insulation from price downturns on standard cash crops (e.g., wheat) (Miller et al. 2015).
- Pulse crop rotations can aid production of subsequent wheat crops.—Research shows that wheat crops benefit from a preceding legume pulse crop through the addition of soil organic matter leading to conservation of soil moisture and the addition of nitrogen (Miller et al 2002; Miller et al 2003; Cutforth et al 2007). Benefits, which improve resilience, include improvements in soil fertility and water-use efficiency, plus disruption of weed, pest, and disease life cycles. This finding has encouraged incorporation of pulse crops into rotations with wheat (Long et al. 2014), replacing summer fallow years. Miller et al. (2015) also show that in a wheat-pea cropping system, producers can reduce the amount of nitrogen that they apply, but in the long run maintain similar profits as a wheat-fallow system and reduce uncertainty around those profits.
Depending on the farmer’s perspective, the increase in pulse crop acreage might reflect a response to observed climate change, an adaptation in anticipation of expected climate change, or simply a management change in the interest of soil health. To determine if climate change is playing a role in these crop selections, we compared the relationship between acres of lentils planted, prior-year price, and prior-year precipitation in north central and northeastern Montana (where prior-year precipitation represents a direct climate driver). When the regions were assessed independently, prior-year price appeared to be a strong predictor of crop selection whereas previous-year precipitation was not. This relationship suggests that variables other than Montana climate, such as market demand and/or climate forces outside Montana, may be more important in determining a farmer’s decision to plant a specific crop. Agricultural traditions within each region may also influence crop-selection shifts.
Regardless of each farmer’s reasons for adding pulse crops, this diversification provides benefits to soil health and helps build market resilience in the face of climate change (Zentner et al. 2002; Miller et al. 2015). Still, it should be recognized that pulse crops, like commodity grains, will experience a combination of climate change effects, some of which may counteract each other. For example, heat stress and pathogens may increase with a rise in temperatures, resulting in a decrease in production. However, more atmospheric CO2 is predicted to increase crop biomass and subsequent yields, and reduce water use by allowing plant stomates to open over shorter periods, thus assimilating the same amount of atmospheric CO2 while conserving moisture (Cutforth et al. 2007). To further complicate matters, grain protein can decrease under high CO2, demanding increased nitrogen fertilizer to maintain quality (Kimball et al. 2001). Optimum crop selections and rotation planning are not trivial to optimize under such changing climate conditions.
Agronomists in the northern Great Plains have made significant progress over the last 15 yr encouraging the use of pulse crops, green manure, and cover crops to replace fallow land and reduce soil erosion (Miller et al. 2002; Tanaka et al. 2010; Nielsen et al. 2016). Studies show that soil moisture retention in most years did not significantly decrease with the presence of these crops (Miller et al. 2006; Miller and Holmes 2012), suggesting that this revenue-generating crop can replace a fallow year without incurring a moisture deficit. This beneficial opportunity, however, may not persist as evaporative and transpiration demands increase with projected warming temperatures under climate change.
The variable nature of climate change effects on pulse crops is leading to a variety of research approaches to enhance their versatility. For example, breeding varieties for early flowering and maturity takes advantage of earlier springs and avoids late-summer drought; and breeding to produce cold-tolerant pea and lentil varieties allows fall seeding. Fall seeding, in particular, enables improved seedling establishment when field conditions are warmer and drier, creates more balanced field labor requirements between fall and spring, and improves yield by avoiding high temperatures that quicken maturity (Chen et al. 2006; Cutforth et al. 2007).
Irrigation demand and supply
Irrigated agriculture in Montana involves a variety of crops (e.g., hay, grains, pasture, vegetables) in diverse settings, so generalizations about how a changing climate will affect demand are difficult. Furthermore, hay, pasture, and to a lesser degree grains are vital components of the livestock industry in Montana, so the implications of irrigation demand and supply extend well beyond crop yields alone. See the section of livestock for further discussion of these relationships. The Water chapter describes the basic hydrology of irrigation water supply, but superimposed on that are combined effects of increasing temperatures and dynamic cropping conditions. For example, longer growing seasons prolong water demand, and with earlier snowmelt and less water available late in the growing season, irrigated hay production is already, and will likely continue to be, constrained.
The difference between irrigated and non-irrigated hay production in tons/acre has increased over time since the 1960s (Figure 5-6). Since hay is made up of grasses and broadleaf species, comparing the production in water limited (non-irrigated) versus unlimited (irrigated) conditions is a way to estimate impacts of a warming climate on hay and forage production. The increasing rate of difference between irrigated and non-irrigated hay from northwest Montana to southeast Montana is correlated with a wet-to-dry gradient further suggesting a climate impact on productivity of animal forage. If one assumes water use efficiency to be constant over the mixed species hay crop, there is a climatic mechanism that explains the proportionally greater growth when the crop is irrigated: increased transpiration demand on the non-irrigated plants resulting in decreased productivity. Increased water use efficiency with improved irrigation technology could confound these results, as could increased atmospheric CO2 fertilization. However, there is little evidence that water use efficiency in hay production has increased significantly over time in the western US with improved irrigation technology (Schaible and Aillery 2012). The proportion of alfalfa in the total-hay-production statistic decreases from northwest to southeast Montana, which should increase the water use efficiency of the crop (Hendrickson et al. 2013). However, the opposite result appears to be the case, further implicating the role of climate or a climate/CO2 interaction. The major concern with this trend is not just its impact on hay but also on rangeland native plant communities that are relied upon for livestock production for a large proportion of each year. Thus, to produce the same amount of hay in the future as today, Montana may increasingly seek to rely on irrigation. Yet at the same time less water may be available for irrigating hay given projections of reduced mountain snow pack (see Water chapter).
Hay Production |
![]() |
Figure 5-6. The difference between irrigated and non-irrigated hay production (i.e., irrigated hay production - non-irrigated hay production), which includes grass and alfalfa (USDA-NASS 2015). |
Climate change is likely to exacerbate the relationship between increasing irrigation demand due to increasing temperatures and diminishing irrigation water supply from depleted groundwater and surface water storage (see Water chapter). Diminishing water supply will impact other crops beyond hay. For example, irrigated grain crops—including sugar beets, dry beans, potatoes, barley, wheat, and corn—will face analogous constraints of greater need for irrigation with less available water.
The capacity for farmers to modify crop selection and timing on an annual basis and respond to short-term weather fluctuations (year to year) makes it difficult to discern climate-change impacts in recent trends. But one thing is relatively certain: as climate changes and humans respond, the importance of irrigation to agriculture in Montana will not diminish (and, indeed, may grow).
Given the economic importance of highly developed crop irrigation districts in Montana and their susceptibility to climate change impacts, updates of infrastructure and careful management will be essential as impacts from changing climate become more pronounced with time. Such areas include the Bitterroot Valley, southern Flathead Valley, Beaverhead Valley, Fairfield Bench (Sun River Valley), Gallatin Valley, Musselshell Valley, Clarks Fork of the Yellowstone, the lower Yellowstone, and Milk River Valley. Most of these irrigation districts were constructed in the early 1900s and currently support high-value crop production, including market garden vegetables, alfalfa seed, malt barley, sugar beets, dry beans, potatoes, soybeans, corn, and hay.
Other large-scale production crops: sugar beets, potatoes, and organic grains
Sugar beets, seed potatoes, and organic grains are the three major crops grown by Montana farmers at substantial economic returns, but constitute much less acreage than the conventionally produced, major commodity small grains that dominate Montana farmland (e.g., wheat and barley; Table 5-1). Each of these three crops is economically significant in one or more agricultural regions within the state.
As with grains, no Montana-specific, peer-reviewed literature exists regarding climate change effects for any of these crops. For the crops under discussion in this section (and beyond), some climate changes may be favorable in the short term but may become increasingly disruptive as they persist and cross threshold levels. The exact timing and nature of these effects will vary, depending on such things as crop variety selection, farm microclimates, and market perturbations.
Sugar beets and potatoes.—Researchers have studied the underlying climate controls for sugar beet and potato production over large regions (Tubiello et al. 2002; Jones et al. 2003; Haverkort and Verhagen 2008; Qi and Jaggard 2008). Although these studies do not apply specifically to Montana, they do illustrate several guiding principles that are useful in interpreting change and formulating expectations anywhere, including Montana. While crop growth models indicate that increased atmospheric CO2 levels will increase crop growth potential, the accompanying suite of growth variables will play out differently in different locations given the changing temperatures and precipitation patterns expected (see Climate chapter). Based on the information in these studies, we might expect to see the following in Montana:
- In more northerly locations, such as Montana, longer frost-free seasons may assist growth, but increased volatility and extreme events may reduce yields.
- Increased precipitation can increase or decrease yields in certain seasons, but in some situations, increased fall rain may also hinder harvest and diminish the quality of sugar beets and potatoes.
- Timing and quantity of irrigation are particularly important for both sugar beets and potatoes, but requirements are not the same for both crops.
- As temperatures rise, Montana’s seed potato industry, famed for low prevalence of disease, will likely face more disease pressure, particularly in areas where precipitation also increases.
The preceding list suggests that in the short term, sugar beet and potato production may face different responses to climate change, but in the long run water limitations due to rising temperatures and other climate-induced stresses could pose substantial challenges.
Organic grains.—Organic grains represent a small fraction (<1%) of the total acreage dedicated to conventional small grains, such as wheat and barley. Nevertheless, Montana produces more USDA-certified organic wheat—$27 million in 2015 (USDA-NASS 2015)—than any other state, and the acres planted with organic wheat continue to increase.
Organic grains are expected to be subject to many of the same climate-change challenges as small grains (discussed previously). However, the demand for organic products is less price sensitive than for conventional grains, and their production is not distributed globally as for conventional small grains (Bonti-Ankomah and Yiridoe 2006). They are marketed through a combination of direct and wholesale channels operating outside the mainstream grain transport and sales infrastructure. Many of the complications associated with price and input cost uncertainty in conventional agriculture are diminished or different for organic production.
Management for climate change and management for other dimensions of farm health are increasingly converging. Indeed, organic farming includes several practices that build resilience in ways that may be instructive for other sectors of Montana agriculture adapting to climate change. Such organic farming practices include a) prioritizing cover-cropping for soil health, moisture retention, and pest management; b) direct ties to food processing and retailing to reduce exposure to intermediaries; and c) increased emphasis on seed diversity. The issues embedded in seed diversity—that is the local adaptation and availability of diverse crop varieties—are important to the organic community and gain broader attention as plants become more stressed due to a changing climate. These practices are not new and were not initially driven by climate change, although the principles behind organic farming have always included promoting diversity and soil health. Many farms are increasingly incorporating organic farming practices that build resilience, regardless of their organic status. This trend will likely continue and help Montana adapt to climate change.
Specialty Crops.—Specialty crops are defined in law as “fruits and vegetables, tree nuts, dried fruits and horticulture and nursery crops, including floriculture” (USDA-NIFA undated). With the exception of tree nuts, Montana agriculture includes crops from each of these categories, although the acreage and revenues of specialty crops pale in comparison to those of commodity grains, livestock, sugar beets, and seed potatoes (Table 5-1). We focus here on fruit and vegetables, as food crops, although the principles likely apply to all specialty crops.
Longer periods of frost-free days and warmer temperatures overall improve growing conditions for most fruit and vegetable crops. Plant hardiness expectations, based on USDA data, suggest such changes may be underway in Montana, although it is not possible to identify farm-scale microclimate changes because the analysis is national and based on 30-yr temperature averages (NCA 2014b).
A 2015 USDA report (Brown et al. 2015) on how climate affects agriculture delineates the sensitivities of specialty crops to many climate components (e.g., temperatures, atmospheric CO2 levels, water supply, cloud and light conditions, high winds and other extreme conditions). The report includes generalizations for annual versus perennial specialty crops and notes that specifics of management and microclimates will govern yields and profits. Some climate change effects will be beneficial for plant growth (e.g., elevated CO2 concentrations and longer frost-free seasons), while others will be detrimental (e.g., plant damage due to extreme events, increased weed growth, new or expanded pests and diseases).
While fruit and vegetable agriculture is not a dominant sector in Montana, these crops are a key part of the Montana food market. They are typically more susceptible to erratic weather associated with climate change than are commodity crops. Small farm size, high per-acre crop values, and diverse marketing options can offer flexibility for specialty crops in many respects, but the challenges of perishability and intensive labor requirements counteract those benefits.
Fruits and vegetable crops are almost always dependent on irrigation in Montana. Paradoxically, this dependence is both a vulnerability and a strength. Dependence on irrigation represents risk. However, the use of drip-irrigation and intensive farming methods can enable small-scale food production where it is infeasible for more extensive commodity crops to adopt drip irrigation. Therefore, specialty crops represent a potential adaptation for farmers as climate changes. Climate changes outside Montana—particularly in California, where drought, fire, and competition with burgeoning human populations—threaten to limit national supplies of many fruits and vegetables. Such changes may amplify demand for Montana-grown crops.
CLIMATE CHANGE EFFECTS ON LIVESTOCK
Livestock production directly and indirectly relies on, and influences, virtually all other sectors of agriculture in Montana. The nature of the relationship varies geographically within the state, and sometimes varies within single counties and neighborhoods, depending on microclimates and cultural influences. Thus, the differential effects of climate on irrigated hay production, dryland hay production, native or non-native rangeland and pasture resources, and ultimately on feeds used for livestock finishing and backgrounding (grains, pastures and/or harvested forages) all play into livestock agriculture in different ways and at different times in the production cycle. Direct effects of climate on the animals themselves are also expected and discussed last in this section.
Forage and feed
To understand how climate plays out in the livestock industry and why climate signals are difficult to extract, it is necessary to recognize the industry structure in Montana. For cow-calf producers who rely substantially on irrigated hay production, irrigation supply issues may dominate climate change considerations, whereas for producers who rotate dryland hay with commodity crops, associated price/supply dynamics may predominate. On the other hand, for many producers grazing on non-cropped pasture and rangeland may largely govern the economics of feeder calf production. Many producers employ a composite of two or more of these feed sources (irrigated or dryland hay, crop residues, cover crops, rangelands), even adapting the relative importance of different feeds from year to year. Demand for Montana hay exports may also be driven by climate changes outside Montana (e.g., drought in southwestern states) and this can also reduce supplies in Montana. Other options for resilient management of ruminants under variable ranch conditions include grazing stockpiled forage, and/or swathed windows and bale-grazing, as well as the use of protein and energy supplements for winter feeding where forage quantity or quality is inadequate. All of these tools are already in use to varying degrees in the region and may become more widely practiced as conditions demand.
On top of this production mosaic, the methods, feed supplies, and marketing decisions used to bring meat animals to finish weight after initial calf or lamb production impose an additional suite of climate-dependent variables on livestock economics and ecology. The majority of commercial livestock producers in Montana market calves and lambs to buyers who will finish the animals (in and out of state) in grain- and/or forage-based feedlots. Other producers retain ownership during feedlot finishing, and still other producers are increasingly focused on grass-finishing of ruminants (i.e., cows, sheep, bison, goats) and direct sales. Although the grass-finishing sector is presently a small portion of the total livestock economy in Montana (and elsewhere), it is emerging as a focal topic in some circles as expectations of future climate change attract more attention. Commercial hog and poultry production, in contrast, are more exclusively dependent on feed grains. Dairies combine hay and grains for feed so their vulnerabilities to climate change are mixed. Hogs and poultry may be less vulnerable to climate change compared to ruminants as long as grain supplies are stable. However, they lack some options for flexible feeding that ruminants can exploit if grain supplies destabilize (e.g., adaptation to various forage types and locations).
Forage quantity and species distribution
Given the multi-layered structure of livestock production outlined above, efforts to analyze the influence of climate change on the forage end of the livestock cycle consider both species distribution and forage growth with respect to temperature, precipitation, and CO2 concentration. The countervailing forces of rising temperatures (which may eventually lead to plant stresses) versus increases in CO2 and/or precipitation (which enhance plant growth) will almost certainly alter forage productivity and community composition over time. Models predict that native vegetation production will increase (Morgan et al. 2011; Mueller et al. 2016) but forage quality will decrease (Milchunas et al. 2005). However, a range of experimental and modeling studies demonstrate that the net effects will vary depending on the particulars of local species composition, climate variables (including animal heat stress), and range or pasture management (Izaurralde 2011; Reeves et al. 2014; Mueller et al. 2016; Reeves and Bagne 2016).
The timing of precipitation is an especially important factor affecting forage plant growth and rangeland plant communities (Fay et al. 2002; Heitschmidt et al. 2005; Bates et al. 2006; Prevéy and Seastedt 2014; Hamilton et al. 2016). Late winter snows are the driver in one eastern Idaho location (e.g., Dagliesh et al. 2011), whereas April-to-June rains are key in a Montana rangeland site. Experimental work corroborates the importance of timing (Heitschmidt et al. 2005). Given projections of small but significant precipitation changes in some parts of the state (see Climate chapter), we can expect that forage patterns will be region- and season-specific. In the long-term, as rising temperatures increase evapotranspiration, heat stress may overtake temporary benefits of well-timed precipitation and CO2 fertilization. The local details will matter in determining both the rate and severity of such forage losses, and we cannot generalize statewide.
In addition to the direct temperature/moisture considerations for rangelands and hay discussed above and in the crop subsection on irrigation demand and supply, three additional forage-related topics are connected to climate’s influence on agriculture. These factors may increase in importance as climate change proceeds, as follows.
- Reductions in Conservation Reserve Program acreage increase livestock producers’ vulnerability to climate-induced supply fluctuations.
- The increasing use of cover crops for various purposes (soil management, pollinator enhancement, other crop rotation goals) can also augment grazing opportunities for livestock producers. Although this currently represents only a small fraction of total grazing in Montana (USDA-FSA 2016), cover crop grazing may become an increasingly important tool for building resilience as climate change continues.
- Increased risk of grassland fire may intermittently threaten forage supplies in Montana, particularly where late season heat and aridity follow early spring rains that build up unusually ample grassland fuels. The vast fires that have recently afflicted portions of the southern Great Plains (spring 2017), demonstrate the potential for catastrophic events that may alter the economic conditions for affected ranchers for the foreseeable future. If such events persist and/or expand in the Great Plains, south or north, reverberations in the livestock industry may be profound.
Empirical data on forage quality
Climate change effects will simultaneously alter forage quality, along with quantity and species distribution, and these components affect animal nutrition. Craine (2010) acknowledges the difficulties of predicting forage quality shifts with climate change and takes a composite, empirical approach to evaluating cattle nutritional stress. The paper reports on decreases in crude protein and digestible organic matter over 14 yr, based on 21,000 cattle fecal samples across the US. By correlating these data with temperature and precipitation data associated with sampling locations, Craine (2010) infers that temperature increases will cause forage decline overall and that increased precipitation in some areas will be unlikely to compensate for declines in forage quality. On this basis, nutritional stress is likely to be seasonally focused in the form of mid-summer growth slumps, and/or late-season quality reduction in forages. Outcomes will depend on both local weather variability and forage management techniques (St-Pierre et al. 2003).
Implications for resilience
Forage studies and other research on tillage practices, moisture retention, carbon storage, and other climate parameters remind us that a climate assessment must acknowledge that not only does climate affect rangelands, but broad expanses of rangeland also may affect climate (Retallack 2013). Grasslands and their organic-rich soils can mitigate rising temperatures by serving as carbon reservoirs (e.g., Retallack 2013). Enhancing grassland production through active management, burning, and grazing rotations may become important parts of resilience strategies in the future.
The preceding discussion of the climate-forage connection is not comprehensive, but reflects a diversity of research approaches to detect climate change effects on livestock feed quality and quantity, as well as the shortage of Montana-specific publications. The grain component of livestock feeding programs is covered in the crops section of this chapter. The connections to irrigation practices and global grain supplies, to Conservation Reserve Program land, to cover-cropping practices, and to fire risks alluded to in this section and elsewhere in this assessment are all reminders that the interdependence of livestock and crop agriculture will likely loom large as Montana experiences the cumulative effects of climate change.
Heat stress
Examples of mechanisms and patterns, summarized below, help explain why there is such variability, and also reveal the avenues for building resilience in livestock operations to help mitigate animal stress. Heat stress affects ruminants through numerous physiological mechanisms (Nardone et al. 2010; Sevi and Caroprese 2012), and the timing, genetic make-up, and other variables determine the severity of the impacts (Bohmanoa et al. 2008; Bradshaw and Holzapfel 2008; Baumgard and Rhoads 2012). Relative humidity particularly influences the apparent or felt temperature, commonly expressed as the heat index, affecting livestock stress. Increased water vapor is expected to accompany increases in temperature (IPCC 2013), and as a result heat stress increases are compounded. In addition, heat impacts grazing animals differently than animals in confinement settings (Parsons et al. 2001; Turnpenny et al. 2001). Mu and McCarl (2011) predict that pasture use will increase relative to cropland based on modeling a combination of forage and animal response factors. Allred et al. (2013) suggest that native grazers may be better suited in the northern Great Plains than domestic cattle, citing different grazing behaviors in arid conditions.
Financial costs of heat stress are expected to increase in northern states, such as Montana, as summer temperatures rise. Based on comparisons with the southern US, where heat stress is already a significant cost estimated at a total of $2.4 billion annually for all livestock sectors (St-Pierre et al. 2003), we can infer that the costs of heat stress in Montana will become significant as the number of days above 90°F (32°C) increases. Despite consensus on these general points, an absence of specific projections once again characterizes the discussion, due to the complex mixture of microclimates, human agency, seasonality factors, genetics, and more. In addition, considerable evidence suggests that heritability indices are high and that genes for heat- and cold-tolerance are different. Thus, simultaneous selection for hot and cold conditions within breeds is potentially feasible in states like Montana (Howard et al. 2014), and discussion of these issues is already underway (e.g., Lemme et al. 2010).
Discussion of livestock feeding modes (grains versus forage) and animal management (intensive versus extensive) will arise as agriculture develops strategies for responding to a shifting climate. Ultimately, finding the optimal combinations of finishing methods for ruminant livestock (feedlot grains and/or forage strategies) will govern much of the economics and resilience of the livestock industry.
CLIMATE CHANGE EFFECTS ON POLLINATORS, DISEASE, PESTS, AND WEEDS
In this section, we look at potential climate change impacts on agriculturally significant pollinators, crop and animal diseases, and weeds and assess the implication of those effects for Montana agriculture.
Pollinators
The crucial role of pollinators (both commercial honeybees and wild pollinators) to agriculture, including in Montana, is undisputed. Researchers expect climate change to influence pollinators, primarily through elevated temperatures (Aizen et al. 2009).
No literature exists to describe climate change impacts on pollinators specifically in Montana. The majority of research on pollinators in agroecosystems has focused on such topics as habitat fragmentation, agrichemical use, and crop distribution, but less explicitly on climate change (Aizen et al. 2009). Efforts to examine long-term trends related to pollinators and associated with climate change are becoming more prevalent. Although that work has largely focused on non-agricultural systems, it is nonetheless instructive. Examples follow:
- In a warming climate, the timing of activity (i.e., phenology) of plants and pollinators is expected to shift, but these shifts may not be synchronized with one another (Burkle and Alarcon 2011; Burkle et al. 2013; Rafferty et al. 2013).
- Burkle and Runyon (2016) examine possible mechanisms underlying changes in pollinator behavior resulting from climate change and report that volatile organic compounds emitted by flowering plants are a primary pollinator attractant. Those floral volatiles may increase with drought, which is likely to be exacerbated, when and where it occurs, by climate change in Montana. However, prolonged water stress may in fact reduce production of floral volatiles, so climate effects on pollinators through that signal may be non-uniform.
- Focusing specifically on North American bumblebees, Burkle and Alarcón (2011) identify the main potential threats from climate change, including shifts in the timing of cues that initiate life history events, community interactions, and habitat growth. These threats could be applicable to other species.
- Otto et al. (2016) describe land-use changes (e.g., a major increase in acreage dedicated to corn and soybeans) in the northern Great Plains that are reducing suitable locations for honeybee colonies. Analogous crop shifts in Montana, potentially linked to climate change, could be similarly significant.
- Climate change is expected to influence the foraging activity, body size at maturity, and life span of wild pollinators (reviewed in Scaven and Rafferty 2013). Large-bodied pollinators are expected to be better able to thermoregulate (Bishop and Armbruster 1999) but are more likely to overheat than small-bodied pollinators (Heinrich 1993), which could influence foraging behaviors (Willmer 1983; Cooper et al. 1985). Warmer temperatures are expected to result in smaller adults with shorter lifespans (Bosch et al 2000; Bosch and Kemp 2003), which can influence pollinator effectiveness (Sahli and Connor 2007).
Montana is the second-largest honey-producing state in the US (USDA-NASS 2015). Each year beekeepers move Montana hives across the country to provide pollination services to other agricultural regions. Many Montana honeybee hives spend winter months in intensive agriculture regions (e.g., California almond orchards) before returning to a variety of forage-, prairie-, and grain-dominated landscapes in Montana. Thus, even if specific climate change effects on pollinators in Montana do materialize (or have already), they may be difficult to distinguish from non-local stressors.
The role of native pollinators in Montana agriculture is often underestimated. That role can be diverse and robust even as reports of commercial honeybee declines dominate headlines (Ollerton et al. 2012; Garibaldi et al. 2013; Rader et al. 2016). Like commercial honeybees, native pollinators are vulnerable to a variety of drivers, not just climate change. Thus, discerning a discrete climate-change signal is similarly challenging. Research may more readily detect climate-influenced patterns for native pollinators, however, since they are not transported around the country.
Wild pollinators by themselves can sufficiently pollinate certain crops (Kremen et al. 2002; Winfree et al. 2007), and wild pollinator diversity is the most important factor in stable pollination services to crops, regardless of whether honeybees are also present (Kremen et al. 2002; Klein 2009; Garibaldi et al. 2011; Rader et al. 2016). Thus, enhancement of native pollinator habitat (floral and nesting resources, natural or managed lands) represents an important avenue to support current and future pollination services in Montana agriculture (L. Burkle, Montana State University, personal communication, unreferenced). As the quality of some agricultural lands decline with climate change and more land comes under cultivation and development (Oleson and Bindi 2002), natural and semi-natural habitat will become more threatened. This potential situation only reinforces the importance of such enhancements for the maintenance of healthy wild pollinator communities (Garibaldi et al. 2011).
Crop diseases
Attributing fluctuations in crop disease directly to climate shifts is again uncertain and complex (Anderson et al. 2004; Garrett et al. 2011). A number of researchers have described plant disease expectations considering climate change variables, but without a Montana-specific focus (Canto et al. 2009; Chakraborty and Newton 2011; Garrett et al. 2011; Luck et al. 2011).
Still, we do have significant knowledge of the ecology of economically important crop diseases in Montana and we expect climate shifts will change crop disease impacts (e.g., yield losses, crop quality). Several examples follow:
- Stripe rust (Puccinia striiformis Westend), a wheat rust disease found in cooler environments, can lead to substantial yield loss. Farmers often apply preventative fungicide to susceptible wheat varieties, a cost that reduces net returns. Some strains of stripe rust are more aggressive at higher temperatures, some can survive winter conditions, and some can overcome the genes bred into the wheat to make it resistant to strip rust (i.e., termed a resistance gene). Thus, ongoing monitoring will be necessary.
- Wheat streak mosaic virus is a disease caused by a virus carried by the wheat curl mite (Aceria tosichella) and is widespread in north central Montana. Vector survival and reproduction of the virus increase when fall frosts are late and winters mild (thus causing greater impact). In addition, the genetic resistance that is currently present in some wheat varieties breaks down at high temperatures, thus eliminating that resistance strategy. Tillage practices, which can change soil moisture and temperature, may be necessary to reduce virus persistence and spread in no-till or low-till systems.
- Most foliar or leaf spot diseases (e.g., tan spot, septoria) are caused by fungi and will increase if farming practices tend toward more stubble on the ground, and moisture retention is enhanced as a strategy for coping with a warming climate.
- Insect and mite-vectored diseases, such as potato virus Y, barley yellow dwarf, wheat streak mosaic virus complex, and aphid-vectored pea viruses, may be enhanced if temperature changes lead to earlier migration or improved overwintering of vector populations of aphids.
Due to production goals, new crop varieties cannot always be substituted as a response to increased pathogens. The dominant approach for managing crop pathogens is instead, as in several examples above, breeding resistance into crops. One key question for Montana agriculture is this: Can crop breeding keep pace with changes in pathogen prevalence and migration resulting from climate change? Crop breeding alone may not meet the challenge; other tools, such as crop rotations and other measures typically associated with organic methods, may regain prominence as trends in the mean and extremes become more significant. Another key question is arising as some crop selections are shifting: do changes such as pulse crop expansion and/or increased corn acreage in some portions of the state expose the state’s dominant wheat crop to new disease associations and dynamics (for example, fusarium in corn and/or viruses in pulses)?
Insect pests
Currently available data do not allow a comprehensive analysis of the likely impact of climate change on all commercially significant insect pests. We focus instead on one major insect pest—-wheat stem sawfly (Cephus cinctus Norton)—on Montana’s dominant crop, wheat, to illustrate the mechanisms and principles involved in assessing climate change effects on agricultural pests and their impacts on crop yield or quality. This approach demonstrates that various factors can enhance or degrade a pest’s impact on crops. Climate change impact analyses typically project increasing pest survival and crop damage with increasing temperatures (e.g., NCA 2014a), and wheat stem sawfly (WSS) may well be generally consistent with that pattern, but the following caveats help to show why generalizations across all landscapes in Montana, for all insect pests, are risky.
Several climate-related parameters—including indirect influences by crop, insect, and/or environmental traits—can collectively influence insect-pest outcomes. For example, in the case of WSS:
- Crop host.—Montana cropland has historically been dominated by a near monoculture of wheat associated with a large expanse of fallow land. In the last 10 yr, wheat acreage has averaged over approximately 5.6 million acres (2.25 million hectares). WSS (and some other pests such as orange wheat blossom midge) survive only on cereal crops such as wheat. Thus, WSS has become established in a wheat-dominated landscape, yet now that landscape has already begun to shift toward more diverse cropping, including pulses and oilseeds. That diversity may affect WSS-crop dynamics as crop diversification is expected to increase with climate change, but we cannot yet predict how much.
- Pest/parasitoid life cycle.—WSS is a native species that first adapted to spring cereals from grass hosts when crops were initially grown by western settlers (Anonymous 1946). Records show large increases in host range from grasses to spring to winter wheat (Anonymous 1946; Morrill and Kushnak 1996; Ivie 2001). This shift was accomplished by advancing the date of flight of WSS adults, successfully completing development in early maturing winter wheat crops. Currently, yield losses caused by WSS are greater in winter wheat than in spring wheat. Overall losses in wheat crops due to WSS will increase if climate change leads to more winter wheat acres. Compounding this effect, native killing agents of WSS (e.g., parasitoids Bracon cephi Gahan and B. lissogaster Muesebeck) have shorter life cycles than WSS, allowing for two generations per summer as opposed to the single generation of WSS. The first generation parasitoid attacks younger larvae of WSS and the second attacks the large larvae that are preparing to overwinter. However, the second-generation parasitoid cannot locate larvae if the crop ripens quickly and the larvae are no longer active and have already prepared to overwinter. This condition decreases the success of the second generation and reduces overall effectiveness of biological control on WSS. Surveys show a decline in proportion of second-generation parasitoids of WSS over the last decade (D. Weaver, Montana State University, personal communication, unreferenced).
- Timing of harvest.—Due to progressively earlier harvests and more rapid development of winter wheat crops, we expect that the success of the second generation of parasitoids will differ considerably for winter and spring wheat. Initial data are confirming this expectation (D. Weaver, Montana State University, personal communication, unreferenced).
- Resilience declines/feedback loops.—The decrease in overwintering parasitoids (D. Weaver, Montana State University, personal communication, unreferenced) is a significant concern, because the resilience of the parasitoid population might become exclusively dependent on later maturing grasses on the periphery of wheat fields. These grasses might allow the parasitoids to persist, but at insufficient population levels to continue significant mitigation of WSS.26
Although the particulars of host, insect, and ecological setting demonstrate the hazards of detailed projections, they also reveal plausible avenues for building resilience to help withstand climate change. Strategies, such as increasing crop diversity and rotations, retaining grass habitat strips to enable survival of beneficial parasitoids, and further exploring the survival dynamics of WSS and other pests, offer a range of opportunities for reducing vulnerability to pests. The WSS example (also see sidebar) shows that searching solely for simple relationships between temperature and pest survival may be fraught with uncertainty, not only for projection purposes but for adaptation and mitigation design as well. The details of pest/host dynamics clearly matter for WSS, and it is prudent to expect that they matter for other pests, as well.
The Wheat Stem Sawfly and Climate Change Crop injury due to the wheat stem sawfly (Cephus cinctus Norton) was reported, anecdotally, in 1910 from wheat fields near Bainville Montana (Anonymous 1946). The earliest pest records for this species in Montana are from spring wheat only (Ivie 2001). Since the late 1970s, however, the native wheat stem sawfly has used both spring and winter wheat as hosts (Morrill and Kushnak 1996; Lesieur et al. forthcoming). With the addition of winter wheat as a suitable host, the population dynamics of the wheat stem sawfly have changed. Morrill and Kushnak (1996) estimated that adults emerge from overwintering wheat residue approximately 20 days earlier than historical populations. This increasing suitability of winter wheat for the full wheat stem sawfly life cycle, because of the changing growing season, together with increasing winter wheat acreage, effectively doubles the acreage of wheat that can be damaged by the wheat stem sawfly. Historical (dark shading) and recent (light shading) distribution of WSS in wheat crops in the northern Great Plains of the US. Historical means from first record through 2005, while recent is after 2005 (Bekkerman and Weaver forthcoming).
|
||
Infectious disease in animals
Most analyses of expected effects of climate change in agriculture include changes in disease dynamics (Plowright et al. 2012), but Montana-specific data are rare. As discussed throughout this assessment, the interplay of many variables complicates differentiation of the effects of climate change from other driving factors. Still, some recent outbreaks of livestock/wildlife disease in Montana have invited speculation about climate’s role, although without definitive conclusions regarding causation.
Plowright et al. (2012) explain numerous mechanisms of disease transmission and how they will interact with different species and in different locations. The mechanisms of transmission are influenced by a suite of climate variables, metapopulation structures, and population densities and connectivity. The authors further dissect disease dynamics in terms of host behaviors, parasite life cycles, other seasonal attributes of disease transmission, and stress-mediated susceptibility.
Plowright et al. (2012) describe two examples that help explain climate linkages to numerous ecological attributes directly relevant to Montana agriculture:
- Brucellosis transmission.—Brucellosis transmission among elk near Yellowstone National Park is a function of snowpack (affecting elk herding), as well as duration and seasonality of aggregation (affecting overlap with abortion events). Potential transmission from elk to cattle similarly depends on seasonal circumstances, although human management (e.g., movement or segregation of cattle) may mask climate-associated effects.
- Parasite susceptibility in sheep.—For sheep on St. Kilda, an island in the North Atlantic, increasing temperatures has increased primary productivity. That increased productivity, in turn has led to improved body condition possibly enhancing the sheep’s ability to withstand parasites. Parasitism in Montana sheep flocks may increase in response to elevated temperatures in some seasons and some locations, but the opposite could also occur, as shown in the St. Kilda example.
Weeds and invasive plants
Climate change is likely to impact plant distribution in the northern Great Plains and Rocky Mountain regions (Battisti and Naylor 2008), including that of weeds and invasives. We use the term weeds to refer to plant species that impact crops and separate the designation from invasive plants, which may have or have the potential to impact a broader array of agricultural activities on rangeland and pastureland. Increased expenditures for weed and invasive plant management in response to climate change could have significant economic impact on agriculture (Pejchar and Mooney 2009).
Studies show that increased atmospheric CO2 concentration can drive increased weed growth and reproduction, although precipitation is an important mechanism mediating rangeland plant community response (Weltzin et al. 2003; Ziska et al. 2005; Mueller et al. 2016). Independent of precipitation, the combined effects of atmospheric CO2 concentration and warming increased C3 rangeland grass productivity over time in a controlled experiment in Colorado (Mueller et al. 2016). Conversely, others note that
increased temperatures can result in negative impacts to weeds due to increased evaporative demand (Larson 2016). Additionally, Hellman et al. (2008) note that climate change will likely impact weeds and invasive species by altering their transport and introduction mechanisms, establishment, ecological impact, and distribution, as well as the effectiveness of control strategies.
Under rapid climate change, weeds and invasive plants may have an advantage over desired and native plants because many have evolved to excel at dispersal, establishment, and adapting to new and changing environments (Corlett and Westcott 2013). Still, even an obvious regional shift in a weed species can be difficult to attribute to climate change. For example, jointed goatgrass (Aegilops cylindrical), a major weed in winter wheat, has steadily moved north in the Great Plains (Anderson et al. 2004). That movement might be explained by greater warming in the north. Alternatively, it might be a result of patterns of winter wheat harvest, which moves from south to north and has been a major vector of seed dispersal through passive transport on harvest equipment (Petit et al. 2013).
Impacts of increasing temperatures.—Winter hardiness zones are predicted to move north (Parker and Abatzoglou 2016), and elevational boundaries are likely to increase, based on increasing temperature projections (see Climate chapter).
With increased winter temperatures, weeds with a winter annual life cycle (i.e., plants that germinate in autumn and mature in spring or summer of the following calendar year) are likely to exhibit higher winter survival rates. This positive impact on survival rate will result in ranges expanding to the north and to higher elevation (Bradley et al. 2016). The indirect effects of increased fire frequency may also be important for weeds with winter life cycles, because of their ability to rapidly establish on burned landscapes (Bradley et al. 2010; Taylor et al. 2014).
Summer temperatures in Montana are also projected to increase with notable increases in the number of summer days above 90°F (32°C) throughout the 21st century (see Climate chapter). The increase in summer temperatures can contribute to increased wildfire frequency and intensity by drying fuels (Westerling et al. 2011), resulting in increased habitat for invasive species on rangeland (Alba et al. 2015). In addition, extreme heat during grain filling (i.e., the period of wheat development from pollination to seed production) can reduce crop yield (Lanning et al. 2010), thereby adding to the stress exerted by weeds. Those weeds, in turn, are more likely to be adapted to extreme heat.
Impacts of increased atmospheric CO2.—Elevated atmospheric CO2 generally increases plant water-use efficiency more for C3 than C4 plants. Those added efficiencies can translate to increases in biomass accumulation and reproduction, which can favor weeds over crops and invasive species over native forage species (Weltzin et al. 2003). For example, CO2 enrichment has been shown to enhance the growth of downy brome (Bromus tectorum) in low-elevation desert and shrubland sites (Ziska et al. 2005). If Montana climate shifts to be more like that of the Great Basin (warmer and drier than current conditions), we might expect environments to increasingly be more compatible for Bromus tectorum (see sidebar).
The impacts of climate change, whether direct or indirect, will present a significant challenge for weed and invasive plant managers in the future. Management will need to change—most likely to become more adaptive—under climate change (Prato 2008). Two examples follow.
Impacts to herbicides.—Increased atmospheric CO2 concentration will likely decrease the effectiveness of some herbicides important for maintenance of chemical-fallow between cropped years (Ziska et al. 1999; 2004; Wolfe et al. 2008).
Impacts to biocontrols.—Climate change may alter the effectiveness of biocontrol agents, which is the use of natural enemies to reduce invasive species populations and a popular means of invasive species management on Montana rangeland. Negative impacts from climate change could include mismatches in the life cycles between the biocontrol agent and the targeted species (van Asch and Visser 2007), unexpected disruptions in host food webs, or shifts in host selection, all of which would diminish the efficacy of biocontrol agents (Pearson and Callaway 2003). Alternatively, the impacts of climate change may be positive, for example, by improving over-winter survival of the biocontrol agent, increasing its geographic range, or improving life-cycle match between agent and weed (Hellman et al. 2008).
Projections of Weed Expansion with Climate Change A prime example of a weedy plant predicted to increase in the northern Great Plains and Rocky Mountains is non-native downy brome (Bromus tectorum). It establishes rapidly on disturbed soils and is a dominant weed species in crop and rangeland (Bradley 2009; West et al. 2015; Bradley et al. 2016). Chambers et al. (2007) predict that the high flammability of this winter annual weed will increase wildfire frequency and thereby transform large areas of sagebrush steppe from perennial shrub to annual grass dominance decreasing the land’s forage utility. Other studies suggest that its expansion into the northern Great Plains or Rocky Mountains will depend on rates of warming and drying (Taylor K et al. 2014; Larson 2016). Similarly, Bradley et al. (2009) predict that yellow starthistle (Centauria solstitialis) and salt cedar (Tamarix ramosissima) will expand their range, downy brome (cheatgrass) and spotted knapweed will shift in range (neither increase nor decrease), and the range distribution of leafy spurge is likely to contract under predicted climate scenarios. Clearly, weed responses will be highly variable, even when similar driver variables are at play. Non-native downy brome (Bromus tectorum).
|
||
THE FUTURE OF MONTANA AGRICULTURE
This assessment of climate change effects on Montana agriculture must start with the basic observations that temperatures are rising and precipitation trends are variable across seasons and regions in Montana. However, the joint importance of the natural environment and human and cultural market processes creates multiple layers of uncertainty and interactions that complicate identifying the effects of climate change. Observers both on and off the farm and ranch are recognizing the effects of climate change, even when market intricacies and changeable cropping practices seem intertwined. Longer growing seasons, less irrigation water, earlier grain harvests, lilacs in the farmyard blooming ahead of “normal,” and hayfields that “don’t produce like they used to,” are conveying a consistent long-term message, even when prices, net revenues, and other measures of the farm economy are variable. When we combine the on-farm observations with others beyond the farm gate, like northward-moving ranges of songbird species and shifts in important pollinators, a pattern begins to emerge that is steadier than commodity prices (Chen et al. 2011).
Beyond providing some of the direct climate-driven responses to crops and livestock, a climate assessment for agriculture must also point out the likelihood of some seemingly contradictory expectations. In the short term, some regions of Montana may experience combinations of increased precipitation and milder temperatures and/or longer growing seasons that can lead to both positive and negative on-farm outcomes. For example, atypical early fall rains improve fall grazing and infiltration of soil moisture before frost limits infiltration, but these same rains can impair some grain harvests and/or fall plantings. Pest problems may increase in some regions due to increasing humidity and warmer conditions, but elsewhere some disease issues will likely initially diminish as aridity increases. Thus, the impacts of climate change for agriculture will almost certainly be highly variable, including at the local scale that is of most interest to farmers and ranchers.
In the short and long term, Montana agriculture may experience as much or more impact from climate change outside Montana as it does from direct, in-state effects. This potential exists primarily because commodity markets for grains and livestock have profound effects on markets for Montana’s farms and ranches. This phenomenon is already underway and is likely to increase in significance. For example, drought in India has helped build markets for pulse crops like lentils and dry peas in Montana. In more complex scenarios, climate change effects across the globe can lead to geopolitical disruptions that also alter wheat and beef markets in positive or negative ways for Montana agriculture revenues. This susceptibility to global affairs is not new to Montana agriculture, but climate change will likely amplify uncertainty for producers.
In the long term, the dominant implication of climate projections for agriculture is that change will not remain gradual. The masked and messy shifts that are underway may reach tipping points that enable and/or force rapid, transformational change in our food systems. Many of the crop, livestock, market, and ecological changes referenced in this chapter have been buffered by many things: surplus harvests, crop insurance, disaster assistance, off-farm income, on-farm ingenuity, market flexibility, and the intrinsic resilience of our landscapes. Furthermore, consumer and taxpayer capacity to bolster that buffering capacity, through food prices and taxes to cover agricultural subsidies, is finite. Because of the sources of uncertainty, many described in this assessment, we are not currently very good at projecting the exact timing of such disruptions. The familiar mantra “more research is needed” is almost always valid, but also, in the face of climate change, insufficient and likely tardy. Region-specific climate projections, historical data on crop production, and more extensive analysis of crop responses will improve our understanding of future patterns, but uncertainty will persist.
KEY KNOWLEDGE GAPS
Emerging questions about building an adaptive and resilient agriculture involves several lines of inquiry. It is clear that climate change will influence agricultural decision-making in different ways, and the more we focus on local adaptive practices the higher the likelihood of success. Whether one seeks to tweak existing systems or more radically overhaul them over time, the following questions are relevant for the future:
- Precipitation.—With the high certainty of warming and the lower certainty of future trends in precipitation, how do we develop resilient agricultural practices that prepare for divergent futures?
- Crop and livestock models.—a) How can crop and forage production models linked with climate models provide useful projections to inform agricultural decisions? b) Which models best inform management of livestock under predicted new climates? c) What mechanisms for data acquisition and accessibility allow appropriate climate and production model parameterization?
- Water.—a) When and where will irrigation be most disrupted as temperatures rise and water storage declines? b) How can we modify our methods for water retention, allocation, and efficiency to increase crop and livestock resilience to climate variability?
- Soil carbon.—a) In which systems and regions can improving soil organic matter help build resilience under volatile climate conditions, including severe drought? b) How can grassland protection and restoration help increase resilience to climate changes, as well as be integrated into food production? c) Which agricultural practices will build soil carbon reserves and serve as viable greenhouse gas mitigation strategies?
- Input practices.—a) Can inputs continue to be used as insurance to protect against variation? b) Does dependence on inputs contribute to creating less resilient agricultural systems? c) Can some inputs increase resilience?
- Commodity markets.—a) How can increased value-added production practices reduce dependence on volatile commodity pricing and thereby build resilience? b) When and where do traditional methods for farmer and consumer protection (e.g., crop insurance, government reserves) need revision to more effectively respond to climate-change uncertainty? c) How can revision of commodity market practices and expectations help develop resilience in anticipation of climate-change induced volatility? d) What improvements in enterprise-level financial and risk management strategies are needed to better manage market and production risks?
- Crop and livestock diversity.—a) How can introduction of diversity to cropping and livestock selections and systems help build resilience to climate change? b) In which current homogeneous production systems can diversity be reintroduced without economic loss? c) How may increased agricultural diversity impact quantity and quality of goods produced in agriculture?
- Policy.—a) Which state and national policies influence producer’s ability to adopt practices more resilient to climate change? b) What role can Montana seed providers, food processors and distributors play to increase agricultural resilience in the face of the uncertainty presented by climate change?
- Rural Sustainability.—a) How will agricultural communities be maintained and need to change in response to climate change? b) How will decisions at all spatial and temporal scales need to change to increase resilience to climate change?
NEXT STEPS
This assessment of the potential impacts of climate change on Montana agriculture is a starting point to identify and prioritize the aspects of agriculture that might be most impacted. In many cases, there are already signs of significant response. To develop effective adaptation strategies for agriculture, we must understand the local trends in our agroecosystems. Monitoring the local climate and agricultural responses to climate change is a critical first step in creating meaningful knowledge on which to base management decisions. Localized management decision tools that increase our ability to estimate the impact of different climate scenarios in the face of all the other uncertainties are needed for decision-making. Successful development of these tools should not be limited to research by scientists. An all-hands approach will be necessary to address the interdisciplinary and site-specific implications of the interacting climate change effects that have been touched on in this chapter.
Building resilience to climate change in Montana’s agricultural sector is paramount. Three premises underlie our ability to increase agricultural resilience:
- Montana agriculture has always included, and will probably continue to include, a spectrum of approaches within any given system (e.g., cattle production, grain production, market garden vegetable production), but the relative economic importance of the approaches may change (e.g., global versus local marketing, cropping versus livestock, organic versus conventional). We need to be able to understand the economic and environmental impacts of those changes.
- Defining success for agriculture in the future will entail matters of marketing, food supply, and food quality and access, as well as environmental health and farm net income. Therefore, understanding how these factors interact at different scales in space and time will be essential to maintaining sustainable agriculture.
- Change is inevitable.
CONCLUSIONS
An assessment of climate effects on Montana agriculture is complex because of uncertainties inherent in the timing and manifestation of climate change, and because of complexity in how natural systems, agricultural producers, and market processes will react.
Still, the science is clear: climate change is occurring. No Montana producer is guaranteed the status quo—change is happening, even if we cannot yet unravel all its components. Precise projections need not be a prerequisite for mitigation and adaptation. Instead, maintaining or increasing resilience in Montana’s agriculture system is paramount.
That resilience will most likely come from increased diversity in our agricultural products and practices. Montana agriculture already includes a spectrum of strategies, for example, global and local marketing; cropping and livestock; feed yard and grass finishing; and pulse groups and crop/fallow small grain crops. Under climate change, new strategies—for example, breeding forages that are tolerant to high temperature or crops and livestock that are resistant to pathogens—may be necessary. Likewise, the prominence of each strategy may well change, and with it the relative economic importance to our state.
RECOMMENDED FURTHER READING
Aizen MA, Garibaldi LA, Cunningham SA, Klein AM. 2008. Long-term global trends in crop yield and production reveal no current pollination shortage but increasing pollinator dependency. Current Biology 18(20):1572-5.
Bekkerman A, Brester GW, Taylor M. 2016. Forecasting a moving target: the roles of quality and timing for determining northern US wheat basis. Journal of Agricultural and Resource Economics 41(1):25-41.
Jones RN. 2004. Incorporating agency into climate change risk assessments. Climatic Change 67(1):13-36.
Lanning SP, Kephart K, Carlson GR, Eckhoff JE, Stougaard RN, Wichman DM, Martin JM, Talbert LE. 2010. Climatic change and agronomic performance of hard red spring wheat from 1950 to 2007. Crop Science 50(3):835-41.
Miller PR, McConkey BG, Clayton GW, Brandt SA, Staricka JA, Johnston AM, Lafond GP, Schatz BG, Baltensperger DD, Neill KE. 2002. Pulse crop adaptation in the northern Great Plains. Agronomy Journal 94(2):261-72.
Plowright RI, Cross PC, Tabor GM, Almberg E, Bienen L, Hudson PJ. 2012. Climate change and infectious disease dynamics [chapter]. In: Aguirre AA, Daszak P, Ostfeld R, editors. New directions in conservation medicine. Oxford UK: Oxford University Press. p 111-21.
Sainju UM, Jabro JD, Stevens WB. 2008. Soil carbon dioxide emission and carbon content as affected by irrigation, tillage, cropping system, and nitrogen fertilization. Journal of Environmental Quality 37(1):98-106.
LITERATURE CITED
Aakre D. 2013. Why grow wheat or barley? [slide presentation]. 19 p. Available online https://www.ag.ndsu.edu/smallgrains/presentations/2013-best-of-the-best-.... Accessed 2017 Mar 6.
Aizen MA, Garibaldi LA, Cunningham SA, Klein AM. 2009. How much does agriculture depend on pollinators? Lessons from long-term trends in crop production. Annals of Botany 103(9):1579–88. doi:10.1093/aob/mcp076.
Alba C, Skálová H, McGregor KF, D’Antonio C, Pyšek P. 2015. Native and exotic plant species respond differently to wildfire and prescribed fire as revealed by meta-analysis. Journal of Vegetation Science 26(1):102-13.
Allred BW, Fuhlendorf SD, Hovick TJ, Dwayne Elmore R, Engle DM, Joern A. 2013. Conservation implications of native and introduced ungulates in a changing climate. Global Change Biology 19(6):1875-83.
Anderson PK, Cunningham AA, Patel NG, Morales FJ, Epstein PR, Daszak P. 2004. Emerging infectious diseases of plants: pathogen pollution, climate change and agrotechnology drivers. Trends in Ecology & Evolution 19(10):535-44.
Anderson RL, Hanavan D, Ogg Jr AG. 2004. Developing national research teams: a case study with the jointed goatgrass research program. Weed Technology 18(4):1143-9.
Anonymous 1946. The wheat stem sawfly in Montana. In: McNeal FH. Wheat stem sawfly literature. 1981. Bozeman MT: Montana Agricultural Experiment Station and Montana Extension Service. 10 p.
Asseng S, Ewert F, Martre P, Rötter RP, Lobell DB, Cammarano D, Reynolds MP. 2015. Rising temperatures reduce global wheat production. Nature Climate Change 5(2):143-7.
Bates JD, Svejcar T, Miller RF, Angell RA. 2006. The effects of precipitation timing on sagebrush steppe vegetation. Journal of Arid Environments 64:670–97.
Battisti DS, Naylor RL. 2008. Historical warnings of future food insecurity with unprecedented seasonal heat. Science 323:240–4.
Baumgard LH, Rhoads RP. 2012. Ruminant nutrition symposium: ruminant production and metabolic responses to heat stress. Journal of Animal Science 90(6):1855-65.
Bekkerman A, Weaver D. [forthcoming]. Modeling joint dependence of managed ecosystems pests: the case of the wheat stem sawfly. Ecological Economics, in review.
Bishop JA, Armbruster WS. 1999. Thermoregulatory abilities of Alaskan bees: effects of size, phylogeny and ecology. Functional Ecology 13(5):711-24.
Bohmanova J, Misztal I, Tsuruta S, Norman HD, Lawlor TJ. 2008. Short communication: genotype by environment interaction due to heat stress. Journal of Dairy Science 91(2):840-6.
Bonti-Ankomah S, Yiridoe EK. 2006. Organic and conventional food: a literature review of the economics of consumer perceptions and preferences. Final report to Organic Agriculture Centre of Canada. 59 p. Available online http://www.organicagcentre.ca/researchdatabase/res_food_consumer.asp. Accessed 2017 July 27.
Bosch J, Kemp WP. 2003. Effect of wintering duration and temperature on survival and emergence time in males of the orchard pollinator Osmia lignaria (Hymenoptera: Megachilidae). Environmental Entomology 32(4):711-6.
Bosch J, Kemp WP, Peterson S. 2000. Management of Osmia lignaria (Hymenoptera: Megachilidae) populations for almond pollination methods to advance bee emergence. Environmental Entomology 29(5):874-83.
Bradley BA. 2009. Regional analysis of the impacts of climate change on cheatgrass invasion shows potential risk and opportunity. Global Change Biology 15:196–208.
Bradley BA, Blumenthal DM, Wilcove DS, Ziska LH. 2010. Predicting plant invasions in an era of global change. Trends in Ecology & Evolution 25(5):310-8.
Bradley BA, Curtis CA, Chambers JC. 2016. Bromus response to climate and projected changes with climate change [chapter]. In: Germino MJ, Chambers JC, Brown CS, editors. Exotic brome-grasses in arid and semiarid ecosystems of the western US: causes, consequences, and management implications. Switzerland: Springer. p 257-74.
Bradley BA, Oppenheimer M, Wilcove, DS. 2009. Climate change and plant invasions: restoration opportunities ahead? Global Change Biology 15(6):1511-21.
Bradshaw WE, Holzapfel CM. 2008. Genetic response to rapid climate change: it’s seasonal timing that matters. Molecular Ecology 17(1):157-66.
Brimelow JC, Burrows WR, Hanesiak JM. 2017. The changing hail threat over North America in response to anthropogenic climate change. Nature Climate Change 7:516–22. doi:10.1038/nclimate3321
Brown ME, Antle JM, Backlund P, Carr ER, Easterling WE, Walsh MK, Ammann C, Attavanich W, Barrett CB, Bellemare MF, Dancheck V, Funk C, Grace K, Ingram JSI, Jiang H, Maletta H, Mata T, Murray A, Ngugi M, Ojima D, O’Neill B, Tebaldi C. 2015. Climate change, global food security, and the US food system. 146 pages. doi:10.7930/J0862DC7. Available online http://www.usda.gov/oce/climate_change/FoodSecurity2015Assessment/FullAs.... Accessed 2017 May 10.
Burgess MH, Miller PR, Jones CA. 2012. Pulse crops improve energy intensity and productivity of cereal production in Montana, USA. Journal of Sustainable Agriculture 36:699-718. doi:10.1080/10440046.2012.672380.
Burkle LA, Alarcón R. 2011. The future of plant–pollinator diversity: understanding interaction networks across time, space, and global change. American Journal of Botany 98(3):528-38.
Burkle LA, Marlin JC, Knight TM. 2013. Plant-pollinator interactions over 120 years: loss of species, co-occurrence, and function. Science 339(6127):1611-5.
Burkle LA, Runyon JB. 2016. Drought and leaf herbivory influence floral volatiles and pollinator attraction. Global Change Biology 22(4):1644-54.
Canto T, Aranda M, Fereres A. 2009. Climate change effects on physiology and population processes of hosts and vectors that influence the spread of hemipteran-borne plant viruses. Global Change Biology 15:1884-94.
Chakraborty S, Newton AC. 2011. Climate change, plant diseases and food security: an overview. Plant Pathology 60:2-14.
Chambers JC, Roundy BA, Blank RR, Meyer SE, Whittaker A. 2007. What makes Great Basin sagebrush ecosystems invasible by Bromus tectorum? Ecological Monographs 77(1):117–45.
Changnon Jr SA. 1984. Temporal and spatial variations in hail in the upper Great Plains and Midwest. Journal of Climate and Applied Meteorology 23(11):1531-41.
Chen C, Miller P, Muehlbauer F, Neill K, Wichman D, McPhee K. 2006. Winter pea and lentil response to seeding date and micro- and macro-environments. Agronomy Journal 98:1655-63.
Chen I-C, Hill JK, Ohlemüller R, Roy DB, Thomas CD. 2011. Rapid range shifts of species associated with high levels of climate warming. Science 333(6045):1024-6.
Cooper PD, Schaffer WM, Buchmann SL. 1985. Temperature regulation of honeybees (Apis mellifera) foraging in the Sonoran desert. Journal of Experimental Biology 114(1):1-15.
Corlett RT, Westcott DA. 2013. Will plant movements keep up with climate change? Trends in Ecology and Evolution 28(8):482-8.
Craine JM, Elmore AJ, Olson KC, Tolleson D. 2010. Climate change and cattle nutritional stress. Global Change Biology 16(10):2901-11.
Cutforth HW, McGinn SM, McPhee KE, Miller PR. 2007. Adaptation of pulse crops to the changing climate of the northern Great Plains. Agronomy Journal 99(6):1684-99.
Dalgleish HJ, Koons DN, Hooten MB, Moffet CA, Adler PB. 2011. Climate influences the demography of three dominant sagebrush steppe plants. Ecology 92(1):75-85.
Fay PA, Carlisle JD, Danner BT, Lett MS, McCarron JK, Stewart C, Knapp AK, Blair JM, Collins SL. 2002. Altered rainfall patterns, gas exchange, and growth in grasses and forbs. International Journal of Plant Sciences 163:549–57.
Garibaldi LA, Aizen MA, Klein AM, Cunningham SA, Harder LD. 2011. Global growth and stability of agricultural yield decrease with pollinator dependence. Proceedings of the National Academy of Sciences 108(14):5909-14.
Garibaldi LA, Steffan-Dewenter I, Winfree R, Aizen MA, Bommarco R, Cunningham SA, Kremen C, Carvalheiro LG, Harder LD, Afik O, Bartomeus I. 2013. Wild pollinators enhance fruit set of crops regardless of honeybee abundance. Science 339(6127):1608-11.
Garrett KA, Forbes GA, Savary S, Skelsey P, Sparks AH, Valdivia C, Van Bruggen AHC, Willocquet L, Djurle A, Duveiller E, Eckersten H, Pande S, Cruz CV, Yuen J. 2011. Complexity in climate-change impacts: an analytical framework for effects mediated by plant disease. Plant Pathology 60:15-30.
Hamilton TW, Ritten JP, Bastian CT, Derner JD, Tanaka JA. 2016. Economic impacts of increasing seasonal precipitation variation on southeast Wyoming cow-calf enterprises. Rangeland Ecology & Management 69(6):465-73. doi:10.1016/j.rama.2016.06.008.
Harrison MT, Cullen BR, Rawnsley RP. 2016. Modelling the sensitivity of agricultural systems to climate change and extreme climatic events. Agricultural Systems 148:135-48.
Haverkort AJ, Verhagen A. 2008. Climate change and its repercussions for the potato supply chain. Potato Research 51:223. doi:10.1007/s11540-008-9107-0.
Heinrich B. 1993. The hot-blooded insects: mechanisms and evolution of thermoregulation. Berlin: Springer-Verlag. 601 p.
Heitschmidt RK, Klement KD, Haferkamp MR. 2005. Interactive effects of drought and grazing on northern Great Plains rangelands. Rangeland Ecology & Management 58(1):11-9.
Hellmann JJ, Byers JE, Bierwagen BG, Dukes JS. 2008. Five potential consequences of climate change for invasive species. Conservation Biology 22(3):534-43.
Hendrickson JR, Schmer MR, Sanderson MA. 2013. Water use efficiency by switchgrass compared to a native grass or a native grass alfalfa mixture. BioEnergy Research 6(2):746-54. doi:10.1007/s12155-012-9290-3.
Howard JT, Kachman SD, Snelling WM, Pollak EJ, Ciobanu DC, Kuehn LA, Spangler ML. 2014. Beef cattle body temperature during climatic stress: a genome-wide association study. International Journal of Biometeorology 58(7):1665-72.
[IPCC] Intergovernmental Panel on Climate Change. 2013. Summary for policymakers. In: Stocker TF, Qin D, Plattner G-K, Tignor M, Allen SK, Boschung J, Nauels A, Xia Y, Bex V, Midgley PM, editors. Climate change 2013: the physical science basis; contribution of working group I to the fifth assessment report of the Intergovernmental Panel on Climate Change. Cambridge UK and New York NY: Cambridge University Press. 28 p.
Ivie MA. 2001. On the geographic origin of the wheat stem sawfly (Hymenoptera: Cephidae): a new hypothesis of introduction from northeastern Asia. American Entomologist 47:84-97.
Izaurralde RC, Thomson AM, Morgan JA, Fay PA, Polley HW, Hatfield JL. 2011. Climate impacts on agriculture: implications for forage and rangeland production. Agronomy Journal 103(2):371-81.
Jones PD, Lister DH, Jaggard KW, Pidgeon JD. 2003. Future climate impact on the productivity of sugar beet (Beta vulgaris L.) in Europe. Climatic Change 58(1)93-108.
Kimball BA, Morris CF, Pinter Jr PJ, Wall GW, Hunsaker DJ, Adamsen FJ, LaMorte RL, Leavitt SW, Thompson TL, Matthias AD, Brooks TJ. 2001. Elevated CO2, drought and soil nitrogen effects on wheat grain quality. New Phytologist 150(2):295-303. doi:10.1046/j.1469-8137.2001.00107.x.
Klein AM. 2009. Nearby rainforest promotes coffee pollination by increasing spatio-temporal stability in bee species richness. Forest Ecology and Management 258(9):1838-45.
Kouadio L, Newlands N, Potgieter A, McLean G, Hill H. 2015. Exploring the potential impacts of climate variability on spring wheat yield with the APSIM decision support tool. Agricultural Sciences 6(7):686-98.
Kremen C, Williams NM, Thorp RW. 2002. Crop pollination from native bees at risk from agricultural intensification. Proceedings of the National Academy of Sciences 99(26):16812-6.
Lanning SP, Kephart K, Carlson GR, Eckhoff JE, Stougaard RN, Wichman DM, Martin JM, Talbert LE. 2010. Climatic change and agronomic performance of hard red spring wheat from 1950 to 2007. Crop Science 50(3):835-41.
Larson CD. 2016. An experimental approach to understanding how Bromus tectorum will respond to global climate change in the sagebrush-steppe [MS thesis]. Bozeman MT: Montana State University.
Lemme G, McInnes D, Szumigalski T. 2010. Adapting agriculture to climate variability: executive summary. South Dakota State University Agricultural Experiment Station Circulars. Paper 333. Available online http://openprairie.sdstate.edu/agexperimentsta_circ/333. Accessed 2017 May 10.
Lesieur V, Martin J-F, Weaver DK, Hoelmer KA, Shanower TG, Smith DR, Morrill WL, Kadiri N, Cockrell D, Randolph TL, Waters DK, Bon M-C. [forthcoming]. Phylogeography of the wheat stem sawfly, Cephus cinctus Norton (Hymenoptera: Cephidae): implications for pest management. PLoS ONE, in revision review.
Long JA, Lawrence RL, Miller PR, Marshall LA, Greenwood MC. 2014. Adoption of cropping sequences in northeast Montana: a spatio-temporal analysis. Agriculture, Ecosystems, & Environment 197:77-87.
Luck J, Spackman M, Freeman A, Trebicki P, Griffiths W, Finlay K, Chakraborty S. 2011. Climate change and diseases of food crops. Plant Pathology 60:113-21.
Macadam I, Argüeso D, Evans JP, Liu DL, Pitman AJ. 2016. The effect of bias correction and climate model resolution on wheat simulations forced with a regional climate model ensemble. International Journal of Climatology 36:4577–91. doi:10.1002/joc.4653.
Milchunas DG, Mosier AR, Morgan JA, LeCain DR, King JY, Nelson JA. 2005. Elevated CO2 and defoliation effects on a shortgrass steppe: forage quality versus quantity for ruminants. Agriculture, Ecosystems & Environment 111(1-4):166-84.
Miller PR, Bekkerman A, Jones CA, Burgess MA, Holmes JA, Engel RE. 2015. Pea in rotation with wheat reduced uncertainty of economic returns in southwest Montana. Agronomy Journal 107(2):541-50.
Miller PR, Engel RE, Holmes JA. 2006. Cropping sequence effect of pea and pea management on spring wheat in the northern Great Plains. Agronomy Journal 98(6):1610-9.
Miller PR, Gan Y, McConkey BG, McDonald CL. 2003. Pulse crops for the northern Great Plains. Agronomy Journal 95(4):980-6.
Miller PR, Holmes JA. 2012. Comparative soil water use by annual crops at a semiarid site in Montana. Canadian Journal of Plant Science 92(4):803-7.
Miller PR, McConkey BG, Clayton GW, Brandt SA, Staricka JA, Johnston AM, Lafond GP, Schatz BG, Baltensperger DD, Neill KE. 2002. Pulse crop adaptation in the northern Great Plains. Agronomy Journal 94(2):261-72.
Morgan JA, LeCain DR, Pendall E, Blumenthal DM, Kimball BA, Carrillo Y, Williams DG, Heisler-White J, Dijkstra FA, West M. 2011. C4 grasses prosper as carbon dioxide eliminates desiccation in warmed semi-arid grassland. Nature 476:202-5. Morrill WL, Kushnak GD. 1996. Wheat stem sawfly (Hymenoptera: Cephidae) adaptation to winter wheat. Environmental Entomology 25:1128–32.
Mu JH, McCarl BA. 2011. Adaptation to climate change: land use and livestock management change in the US. Presentation at: Southern Agricultural Economics Association 2011 Annual Meeting; 2011 February 5-8; Corpus Christi TX. Available online https://core.ac.uk/download/pdf/6672623.pdf?repositoryId=153. Accessed 2017 May 10.
Mueller KE, Blumenthal DM, Pendall E, Carrillo Y, Dijkstra FA, Williams DG, Follett RF, Morgan JA. 2016. Impacts of warming and elevated CO2 on a semi-arid grassland are non-additive, shift with precipitation, and reverse over time. Ecology Letters 19(8):956-66.
Nardone A, Ronchi B, Lacetera N, Ranieri MS, Bernabucci U. 2010. Effects of climate changes on animal production and sustainability of livestock systems. Livestock Science 130(1):57-69.
[NCA] National Climate Assessment. 2014a. In: Melillo JM, Richmond T, Yohe GW, editors. Climate change impacts in the United States: the third national climate assessment. Washington DC: US Global Change Research Program. 841 p. doi:10.7930/J0Z31WJ2.
[NCA] National Climate Assessment. 2014b. Shifts in plant hardiness zones [website]. Available online http://nca2014.globalchange.gov/report/appendices/climate-science-supple.... Accessed 2017 Mar 6.
Newton J, Kuethe T. 2015 Mar 6. Changing landscape of corn and soybean production and potential implications in 2015 [online article]. Farmdoc Daily 5:42. Available online http://farmdocdaily.illinois.edu/2015/03/changing-landscape-of-corn-and-.... Accessed 2017 Mar 6.
Nielsen DC, Vigil MF, Lyon DJ, Higgins RK, Hergert GW, Holman JD. 2016. Cover crops can affect subsequent wheat yield in the central Great Plains. Crops & Soils 49(3):51-3. doi:10.2134/cs2016-49-3-15.
Olesen JE, Bindi M. 2002. Consequences of climate change for European agricultural productivity, land use and policy. European Journal of Agronomy, 16(4):239-62.
Ollerton J, Price V, Armbruster WS, Memmott J, Watts S, Waser NM, Totland O, Goulson D, Alarcón R, Stout JC, Tarrant S. 2012. Overplaying the role of honeybees as pollinators: a comment on Aebi and Neumann (2011). Trends Ecology & Evolution 27(3):141-2. doi:10.1016/j.tree.2011.12.001.
Otto CR, Roth CL, Carlson BL, Smart MD. 2016. Land-use change reduces habitat suitability for supporting managed honeybee colonies in the northern Great Plains. Proceedings of the National Academy of Sciences 113(37):10430-5.
Parker LE, Abatzoglou JT. 2016. Projected changes in cold hardiness zones and suitable overwinter ranges of perennial crops over the United States. Environmental Research Letters 11(3):034001. doi:10.1088/1748-9326/11/3/034001
Parsons DJ, Armstrong AC, Turnpenny JR, Matthews AM, Cooper K, Clark JA. 2001. Integrated models of livestock systems for climate change studies. 1. Grazing systems. Global Change Biology 7(1):93-112.
Pearson DE, Callaway RM. 2003. Indirect effects of host-specific biological control agents. Trends in Ecology & Evolution 18(9):456-61.
Pejchar L, Mooney HA. 2009. Invasive species, ecosystem services and human well-being. Trends in Ecology & Evolution 24(9):497-504.
Petit S, Alignier A, Colbach N, Joannon A, Le Cœur D, Thenail C. 2013. Weed dispersal by farming at various spatial scales: a review. Agronomy for Sustainable Development 33(1):205-17.
Pirttioja N, Carter TR, and 48 more. 2015. A crop model ensemble analysis of temperature and precipitation effects on wheat yield across a European transect using impact response surfaces. FACCE MACSUR Reports 6:4-4.
Plowright RI, Cross PC, Tabor GM, Almberg E, Bienen L, Hudson PJ. 2012. Climate change and infectious disease dynamics [chapter]. In: Aguirre AA, Daszak P, Ostfeld R, editors. New directions in conservation medicine. Oxford UK: Oxford University Press. p 111-21.
Prato T. 2008. Conceptual framework for assessment and management of ecosystem impacts of climate change. Ecological Complexity 5(4):329-38.
Prato T, Qiu Z. 2013. Potential impacts of and adaptation to future climate change for crop farms: a case study of Flathead Valley, Montana [chapter in online book]. In Singh BR, editor. Climate change—realities, impacts over ice cap, sea level and risks. Croatia: InTech. doi:10.5772/39265.
Prato T, Zeyuan Q, Pederson G, Fagre D, Bengtson LE, Williams JR. 2010. Potential economic benefits of adapting agricultural production systems to future climate change. Environmental Management 45(3):577-89.
Prevey JS, Seastedt TR. 2014. Seasonality of precipitation interacts with exotic species to alter composition and phenology of a semi-arid grassland. Journal of Ecology 102(6):1549-61.
Qi A, Jaggard KW. 2008. The impact of past and future climate change on sugar beet yield in the UK. Presentation at the 5th International Crop Science Congress; 2008; Jeju, Korea. Available online http://www.intlcss.org/files/icss/congress-proceedings/2008-papers/cs1-s.... Accessed 2017 May 10.
Rader R, Bartomeus I, Garibaldi LA, Garratt MP, Howlett BG, Winfree R, Cunningham SA, Mayfield MM, Arthur AD, Andersson GK, Bommarco R. 2016. Non-bee insects are important contributors to global crop pollination. Proceedings of the National Academy of Sciences 113(1):146-51.
Rafferty NE, CaraDonna PJ, Burkle LA, Iler AM, Bronstein JL. 2013. Phenological overlap of interacting species in a changing climate: an assessment of available approaches. Ecology and Evolution 3(9):3183-93.
Reeves MC, Bagne KE. 2016. Vulnerability of cattle production to climate change on US rangelands. Fort Collins CO: US Department of Agriculture, Forest Service, Rocky Mountain Research Station. General Technical Report RMRS-GTR-343. 39 p.
Reeves MC, Moreno AL, Bagne KE, Running SW. 2014. Estimating climate change effects on net primary production of rangelands in the United States. Climatic Change 126(3-4):429-42.
Retallack GJ. 2013. Global cooling by grassland soils of the geological past and near future. Annual Review of Earth and Planetary Sciences 41:69-86.
Ruane AC, Hudson NI, Asseng S, Camarrano D, Ewert F, Martre P, and 42 more. 2016. Multi-wheat-model ensemble responses to interannual climate variability. Environmental Modelling & Software 81:86-101.
Sahli HF, Conner JK. 2007. Visitation, effectiveness, and efficiency of 15 genera of visitors to wild radish, Raphanus raphanistrum (Brassicaceae). American Journal of Botany 94(2):203-9.
Sánchez JL, Fraile R, De La Madrid JL, De La Fuente MT, Rodríguez P, Castro A. 1996. Crop damage: the hail size factor. Journal of Applied Meteorology 35(9):1535-41.
Scaven VL, Rafferty NE. 2013. Physiological effects of climate warming on flowering plants and insect pollinators and potential consequences for their interactions. Current Zoology 59(3):418-26.
Schaible G, Aillery M. 2012. Water conservation in irrigated agriculture: trends and challenges in the face of emerging demands. Washington DC: US Department of Agriculture, Economic Research Service. Economic Information Bulletin No. 99. 67 p. Available online https://www.ers.usda.gov/webdocs/publications/44696/30956_eib99.pdf?v=41744. Accessed 2017 July 14.
Sevi A, Caroprese M. 2012. Impact of heat stress on milk production, immunity and udder health in sheep: a critical review. Small Ruminant Research 107(1):1-7.
Smith WN, Grant BB, Desjardins RL, Kroebel R, Li C, Qian B, Worth DE, McConkey BG, Drury CF. 2013. Assessing the effects of climate change on crop production and GHG emissions in Canada. Agriculture, Ecosystems & Environment 179: 139-50.
St-Pierre NR, Cobanov B, Schnitkey G. 2003. Economic losses from heat stress by US livestock industries. Journal of Dairy Science 86:E52-E77.
Tanaka DL, Lyon DJ, Miller PR, Merrill SD, McConkey BG. 2010. Soil and water conservation advances in the semiarid northern Great Plains [chapter 3]. In Zobeck TM, Schlessinger WF, editors. Soil and water conservation advances in the USA. Special publication no. 60. Madison WI: Soil Science Society of America. p 81–102.
Taylor K, Brummer T, Rew LJ, Lavin M, Maxwell BD. 2014. Bromus tectorum response to fire varies with climate conditions. Ecosystems 17(6):960-73.
Tubiello FN, Rosenzweig C, Goldberg RA, Jagtap S, Jones JW. 2002. Effects of climate change on US crop production: simulation results using two different GCM scenarios. Part I: Wheat, potato, maize, and citrus. Climate Research 20:259-70.
Turnpenny JR, Parsons DJ, Armstrong AC, Clark JA, Cooper K, Matthews AM. 2001. Integrated models of livestock systems for climate change studies. 2. Intensive systems. Global Change Biology 7(2):163-70.
[USDA Census of Agriculture] US Department of Agriculture Census of Agriculture. 2012. 2012 census publications [website]. Available online https://www.agcensus.usda.gov/Publications/2012/. Accessed 2017 July 14.
[USDA-FSA] US Department of Agriculture-Farm Service Agency. 2016. Conservation reserve program statistics [website]. Available online https://www.fsa.usda.gov/programs-and-services/conservation-programs/rep.... Accessed 2017 July 14.
[USDA-NASS] US Department of Agriculture, National Agricultural Statistics Service. 2015. USDA National Agricultural Statistics Service [website]. Available online https://www.nass.usda.gov. Accessed 2017 May 10.
[USDA-NIFA] US Department of Agriculture-National Institute of Food and Agriculture. [undated]. USDA definition of specialty crop [online report]. 10 p. Available online https://nifa.usda.gov/sites/default/files/resources/definition_of_specia.... Accessed 2017 Mar 6.
van Asch M, Visser ME. 2007. Phenology of forest caterpillars and their host trees: the importance of synchrony. Annual Review of Entomology 52:37–55.
Weltzin JF, Belote RT, Sanders NJ. 2003. Biological invaders in a greenhouse world: will elevated CO2 fuel plant invasions? Frontiers in Ecology and the Environment 1(3):146–53.
West AM, Kumar S, Wakie T et al. 2015. Using high-resolution future climate scenarios to forecast Bromus tectorum invasion in Rocky Mountain National Park. PLoS ONE 10(2):e0117893.
Westerling AL, Turner MG, Smithwick EAH, Romme WH, Ryan MG. 2011. Continued warming could transform Greater Yellowstone fire regimes by mid-21st century. Proceedings of the National Academy of Sciences 108:13165–70.
Willmer PG. 1983. Thermal constraints on activity patterns in nectar feeding insects. Ecological Entomology 8(4):455-69.
Winfree R, Williams NM, Dushoff J, Kremen C. 2007. Native bees provide insurance against ongoing honeybee losses. Ecology Letters 10(11):1105-13.
Wolfe DW, Ziska L, Petzoldt C, Seaman A, Chase L, Hayhoe K. 2008. Projected change in climate thresholds in the northeastern US: implications for crops, pests, livestock, and farmers. Mitigation and Adaptation Strategies for Global Change 13(5-6):555-75.
Zentner RP, Wall DD, Nagy CN, Smith EG, Young DL, Miller PR, Campbell CA, McConkey BG, Brandt SA, Lafond GP, Johnston AM. 2002. Economics of crop diversification and soil tillage opportunities in the Canadian prairies. Agronomy Journal 94(2):216-30.
Ziska LH, Faulkner S, Lydon J. 2004. Changes in biomass and root:shoot ratio of field-grown Canada thistle (Cirsium arvense), a noxious, invasive weed, with elevated CO2: implications for control with glyphosate. Weed Science 52:584–8.
Ziska LH, Reeves JB, Blank B. 2005. The impact of recent increases in atmospheric CO2 on biomass production and vegetative retention of Cheatgrass (Bromus tectorum): implications for fire disturbance. Global Change Biology 11(8):1325–32.
Ziska LH, Teasdale JR, Bunce JA. 1999. Future atmospheric carbon dioxide may increase tolerance to glyphosate. Weed Science 47:608–15.
24 Appendix 5-1 on the MCA website expands on these data to show acres harvested, yield per acre, tons of production, and animal numbers for each of the seven agricultural regions (see below) in Montana.
25 Appendix 5-2 on the MCA website provides a more in-depth discussion of basis and its importance to Montana agriculture.
26 For more detail on the impact of WSS, see Appendix 5-3 on the MCA website.