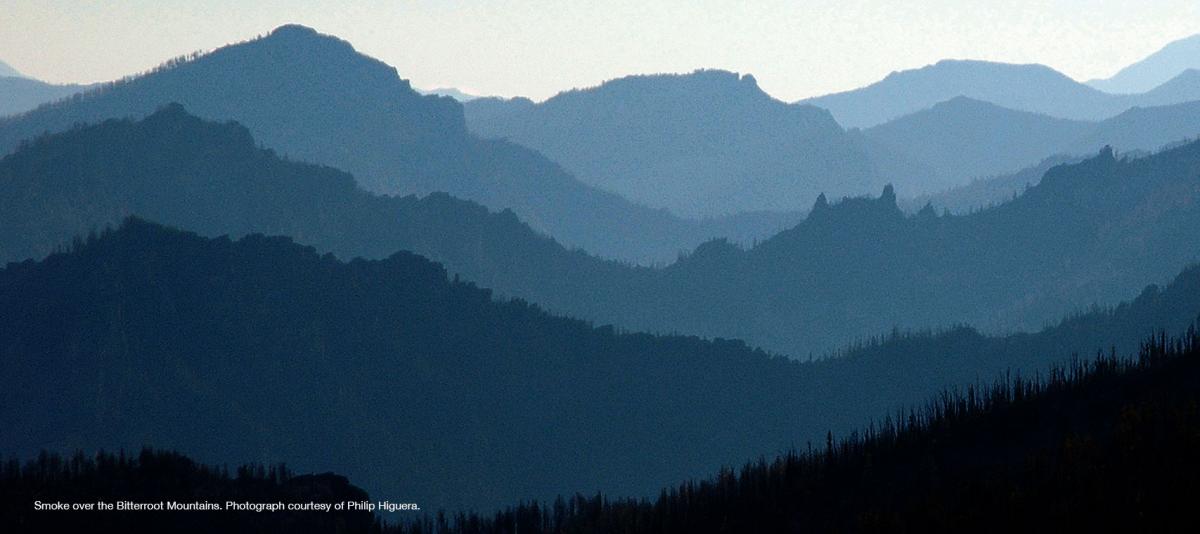
CHAPTER 4
FORESTS AND CLIMATE CHANGE IN MONTANA
Alisa A. Wade, Ashley P. Ballantyne, Andrew J. Larson, and W. Matt Jolly
In this chapter, we interpret how past and projected shifts in climate—as described in the Climate chapter—may influence Montana forests. It is important to note that any potential effects will be spatially and temporally variable, depending on current forest conditions, local site characteristics, environmental influences, and annual and decadal patterns of climate variability, such as the El Niño-Southern Oscillation cycle, which can drive regional weather and climate conditions. Additionally, when discussing drought in this chapter, we are referring to ecological drought as defined in the Drought sidebar of the Climate chapter. The summary of potential climate influences on forest resources provided here are, in part, focused on assisting managers and policy makers develop management responses. Forest managers throughout Montana are key players in maintaining the health of our forests and, ultimately, forest managers will need to consider specific adaptation actions in response to current and potential climate changes. Forest managers also have an important role to play in climate change mitigation via efforts to increase forest carbon storage.
|
KEY MESSAGESIncreased temperatures will have positive or negative effects on individual trees and forest-wide processes, depending on local site and stand conditions, but impacts from increased extreme heat will be negative. [high agreement, moderate evidence]
Direct effects of climate change on individual trees will be driven by temperature in energy-limited forests and moisture in water-limited forests. [high agreement, moderate evidence]
The speed and magnitude of climate change may mean that increased forest mortality and contractions in forest distributions will outpace any gains in forest growth and productivity over the long run, leading to a net loss of forested area in Montana. [medium agreement, limited evidence]
Direct effects of climate change on trees and forests, such as warmer, wetter conditions improving forest productivity or warmer, drier conditions increasing tree mortality, will be secondary to the impacts of altered forest disturbance regimes, such as changes in forest fire behavior and area burned. [high agreement, limited evidence]
An increase in fire risk (i.e., probability of occurrence)—including an increase in size and possible frequency and/or severity (i.e., tree mortality)—is expected in the coming century as a result of a) prolonged fire seasons due to increased temperatures, and b) increased fuel loads from past fire suppression. [high agreement, robust evidence]
Rising temperatures are likely to increase bark beetle survival [high agreement, strong evidence], but climate-induced changes to other insects and forest pathogens are more varied and less certain. [medium agreement, moderate evidence]
Forest responses to climate change may be non-linear and complex due to feedbacks. [high agreement, limited evidence]
There may be a reduction in the amount of carbon stored in forests. [low agreement, limited evidence] |
|
|
BACKGROUND
Forest ownership, communities, and distribution in Montana
In Brief
- There are approximately 23 million acres (9.3 million ha) of forested land in Montana, with the majority publicly owned and in the western part of the state.
- The three most common forest types in the state are dominated by Montana’s most commercially important species: Douglas-fir, lodgepole pine, and ponderosa pine.
- Forest conditions in Montana are varied, and potential impacts from climate change will overlay on existing stresses to forests.
The Montana State Assessment of Forest Resources (MT DNRC 2010) estimates that forested land covers 23 million acres (9.3 million ha) in Montana (Figure 4-1). The majority of Montana forestlands occur in the northwestern climate division (approximately 50%), followed by the southwestern, central, and south central divisions. Additionally, the majority (16.3 million acres [6.6 million ha], 71%) is publicly owned, under the jurisdiction of federal and state agencies (Figure 4-2). Tribal ownership accounts for 5% (1.2 million acres [0.49 million ha]) of forests in Montana. Approximately 5.5 million acres (2.2 million ha) of forestland (24%) is privately owned, with the bulk (4.4 million acres [1.8 million ha], 19% state total) held by more than 83,000 nonindustrial private landowners, and the remainder managed by private industrial forest products companies.
Existing Land Cover in Montana |
![]() |
Figure 4-1. Existing land cover in Montana (Landfire 2012). Gray boundaries delineate climate divisions: 1-northwestern, 2-southwestern, 3-north central, 4-central, 5-south central, 6-northeastern, 7-southeastern (see Climate chapter). |
Percent Forest Ownership in Montana |
|
Figure 4-2. Percent forest ownership in Montana (adapted from MT DNRC 2010). |
There are 10 primary forest types—defined by the dominant tree species in a given area—in Montana as identified and quantified in the Montana State Assessment of Forest Resources (MT DNRC 2010). The three most widespread and commercially important tree species and their direct and indirect sensitivities to climate change are described below.
- Douglas-fir (Pseudotsuga menziesii var. glauca) forests occur in cooler settings, but can tolerate a variety of climate conditions. They are found predominantly in the northwestern climate division (but also in the southwestern and central divisions), on approximately 7 million acres (2.8 million ha) in Montana (Figure 4-3). Douglas-fir trees are moderately tolerant of fire and tolerate drought better than many other species. Douglas-fir forests are subject to damage from western spruce budworm and Douglas-fir beetle, as well as several root diseases (e.g., Armillaria root disease).
Existing Forest Cover Type in Montana
Figure 4-3. Existing forest cover type in Montana (Landfire 2012). Gray boundaries delineate climate divisions (see Figure 2-3). - Lodgepole pine (Pinus contorta var. latifolia) forests occupy approximately 4.9 million acres (2.0 million ha) in Montana (statewide, though primarily in the northwestern and southwestern climate divisions). Lodgepole pine trees grow on moist soils and are highly frost tolerant, but are less drought and fire resistant than Douglas-fir. Still, lodgepole pine forests are well adapted to recolonizing burned areas since lodgepole pine trees reach reproductive maturity at a young age. Lodgepole pine trees are susceptible to mountain pine beetle infestation and resulting mortality.
- Ponderosa pine (Pinus ponderosa) forests are found in drier areas of Montana, predominantly west of the Continental Divide, although east of the Continental Divide ponderosa pine is the dominant commercial timber species. Ponderosa pine forests occupy approximately 3 million acres (1.2 million ha) in Montana, primarily a) mixed with Douglas-fir trees in the northwestern, southwestern, and central climate divisions, and b) as a single species in the south central and southeastern climate divisions. Compared to many other conifers, ponderosa pine trees have deep roots, making them more drought tolerant, and thick bark and high crown, making them more fire adapted. Like lodgepole pine, ponderosa pine trees are susceptible to mountain pine beetle infestation and resulting mortality.
Other conifer forest types found in Montana are spruce-fir forest (primarily composed of Engelmann spruce (Picea engelmannii) and subalpine fir (Abies lasiocarpa), as well as forests dominated by western larch (Larix occidentalis), grand fir (Abies grandis), limber pine (Pinus flexilis), and miscellaneous western softwoods. An additional forest type is composed of hardwoods, including aspen (Populus tremuloides), cottonwood (Populus trichocarpa and P. deltoides), box elder (Acer negundo), bur oak (Quercus macrocarpa), green ash (Fraxinus pennsylvanica), willow (Salix spp.), and birch (Betula papyrifera). Of these hardwood species, cottonwood is the most abundant; it is concentrated in riparian areas of central and eastern Montana.
Urban forests also provide important benefits to quality of life in Montana. However, urban forests, which include many nonnative species, are not a focus of this chapter. Additionally, we will not focus on forest understory species (e.g., shrubs and grasses) despite their importance as wildlife habitat, livestock forage, fire fuels, and socio-cultural importance (see Socio-cultural sidebar). Both urban forests and the forest understory include a vast number of vegetation species, and consideration of species-by-species impacts is beyond the scope of this report.
Potential climate impacts to forests
In Brief
- Forests have evolved, adapted, and transformed in response to natural processes, including disturbance and climate shifts, over the millennia.
- Current levels of atmospheric carbon dioxide are at their highest level in approximately 3 million years, and projected to increase, which will drive climate changes.
- Temperatures in Montana have increased and are projected to continue to rise; there has been no significant change in mean annual precipitation across Montana, but most models project an increase in mean annual precipitation with spring contributions greatest and with slightly reduced summer precipitation. The combination of rising summer temperatures, potential reductions in snowpack (see Water chapter), and decreased or similar summer precipitation will likely make droughts more severe when they do occur. The frequency and severity of extreme events (e.g., drought, extreme hot days) will be enhanced or diminished depending on annual and decadal climate oscillations.
- Individual tree species may prove to be more susceptible to projected changes in climate conditions and associated disturbances, but the actual response of individual species will be spatially complex and dependent on local factors like soils, aspect, water, and nutrient availability.
- There will be direct effects (related to increased temperatures and shifts in precipitation) and indirect effects (changes in disturbance regimes as a result of the direct effects) on forests from climate change.
- Shifts in temperature and precipitation can have both positive and negative direct effects on forest establishment and regeneration, growth and productivity, and mortality. Overall, net impacts are likely to be negative, particularly in water-limited areas.
- Indirect effects from climate change on forest disturbance regimes will likely have the greatest impacts on forest ecosystems, and will complicate patterns of direct effects. Key indirect effects from climate change are likely increases in fire and insect-caused mortality.
- Climate change will directly alter the range of forests with range expansion in some regions and range contractions in other regions. Indirect climate change impacts, such as drought or beetle-induced mortality, will also constrain forest ranges.
- Climate-driven impacts to forest ecosystems may be enhanced or reduced by changes in human land use or management at the forest-stand scale. Forest stand and local site conditions will also greatly influence patterns of climate-driven impacts.
Change is the norm for forests, and over millennia Montana’s forests have transformed and adapted as a result of variations in climate, disturbance, and other natural processes (Whitlock 1993; Brunelle et al. 2005; Power et al. 2011). However, current levels of atmospheric CO2 are higher than those in the last 3 million years (Zhang et al. 2013), and these levels are likely to drive changes to climate (Solomon et al. 2009). Although the magnitude of potential climate change may be comparable to variability experienced in the past, the rate of that change is anticipated to be significantly greater (Diffenbaugh and Field 2013), with substantial implications for Montana’s forests. Montana’s forests will be affected by both direct and indirect effects of climate change. Direct effects are impacts to trees that arise directly in response to changes in temperature and precipitation; indirect effects are secondary impacts, such as increased number of fires associated with warming temperatures, which then affect trees and forests.
In the face of changing climate, forest managers can best maintain stable forest health and product yield by understanding past trends and planning for a range of climate scenarios. The assessments in this chapter are based on the climate trends for which we had sufficient data and climate projections that represent plausible future scenarios, as described in the Climate chapter of this assessment (see Water chapter for snowpack trends and projections) and summarized in Table 4-1.
Climate Metric—Trend and future scenario | Potential direct effects | Potential indirect effects |
Atmospheric CO2 concentrations have increased; projected to increase leading to future warming |
Positive: Increased fecundity, photosynthesis, vegetation water use efficiency, and productivity in some species and locations |
- |
Average temperatures have increased with greatest warming in spring; projected to increase with greatest warming in summer and winter and in the southeast |
Positive: Increased productivity, particularly at higher elevations and cooler areas Negative: Increased plant respiration, evaporation and transpiration; reduced productivity in areas with already high temperatures |
Positive: Increased soil organic matter from increased productivity in some areas; increased nitrogen cycling Negative: Decreased soil organic matter from increased decomposition rates; increased soil acidity; reduced soil (and forest) carbon storage |
Maximum temperatures have increased with greatest warming in spring; projected to increase with greatest warming in summer and winter and in the southeast |
Negative: Increased heat stress, reduced growth and productivity, and increased mortality |
Negative: Increased fire risk |
Days above 90°F (32°C) are projected to increase, with greatest increases in the northeast and south |
Negative: Increased heat stress, reduced growth and productivity, and increased mortality |
Negative: Increased fire risk |
Minimum temperatures have increased most in winter and spring; projected to increase, with greatest increases in January and in the southeast |
Positive: Longer (or at least earlier) growing season; reduced winter mortality Negative: Lower and shorter duration snowpack and shift from snow to rain-dominant precipitation regimes resulting in less water available in summer |
Negative: Increased potential for pathogen and insect survival |
Frost-free days are projected to increase, particularly in the west |
Positive: Longer (or at least earlier) growing season; increased potential for regeneration success; reduced winter mortality |
Negative: Increased potential for pathogen and insect survival |
Growing degree-days are projected to increase, particularly in the southeast |
Positive: Increased opportunities for establishment and regeneration; increased productivity |
Negative: Increased potential for pathogen and insect survival |
Average precipitation has decreased in winter, but no significant change in annual mean precipitation potentially because of very slight increases in spring and fall precipitation; precipitation is projected to increase across Montana, primarily in spring; slight decrease in summer precipitation; variability of precipitation year-to-year projected to increase |
Positive: Increased water availability in spring during critical establishment period Negative: Combined with less available water from reduced and shortened snowpack, drier summers could reduce or shift growing season |
Negative: Increased fire risk |
Number of consecutive dry days shows little projected change, however, increased variability in precipitation suggests potential for more severe droughts, particularly in connection with climate oscillations |
Negative: Reduced establishment, productivity; increased mortality if increased severity of dry spells |
Positive: Reduced disturbance from fungi Negative: Increased fire risk; increased susceptibility to pathogens and insects |
Snowpack has declined substantially; projected to continue to decrease |
Negative: Less available water in summer and potential for increased water stress at same time as highest temperatures |
Negative: Increased fire risk; warmer and drier soils and reduced mycorrhizal activity |
Since 1950, statewide temperatures have shown an upward trend. Although differing somewhat spatially and seasonally, the warming trend is seen across all temperature variables, including annual average, maximum, and minimum temperatures. Similarly, all climate models used in this assessment agree that the average annual temperature in Montana will increase over the next century.
An average statewide increase of 4.5-6.0°F (2.5-3.3°C) is projected for the mid-century and an overall increase of 5.6-9.8°F (3.1-5.4°C) is projected for the end of the century (see Climate chapter for a description of the emission scenarios used to obtain these values). Maximum monthly temperatures are projected to increase, as are extreme heat days (days with temperatures >90°F [32°C]), monthly minimum temperatures, frost-free days, and accumulated growing degree-days.
Changes in precipitation (described in the Climate chapter) are more varied and uncertain. In general, there has been a slight but statistically significant trend of decreasing winter precipitation across the state, averaging -0.14 inches/decade (-0.36 cm/decade) since 1950. Projected future shifts in precipitation are varied, with not all models agreeing on whether precipitation will increase or decrease in Montana. The majority of models suggest a slight increase in total average annual precipitation across the state, largely occurring in spring, particularly in the northwest. The models show less agreement regarding summer precipitation patterns, though a slight majority of models suggest that there may be very small decreases in summer precipitation, particularly in the southeast.
Given these trends and projections for temperature and precipitation, for the remainder of this chapter we consider the impacts of continued warming to Montana forests. In particular, we focus on increasing maximum temperatures in summer and increasing minimum temperatures throughout the winter, with greatest temperature increases in the southern and eastern areas of Montana. We assume a scenario of slightly wetter years on average, with spring precipitation increasing most in the north and western areas, although we assume summers become slightly drier.
Drought has a major impact on forests. An important predictor of future ecosystem drought (defined as ecological drought in the Water chapter) susceptibility is the net balance of water gained through precipitation and water lost through evapotranspiration (the combined effect of water evaporation and the transpiration of water by plants). Predicting how increases in temperature and atmospheric CO2 may shift evapotranspiration is extremely challenging, especially at regional scales such as Montana. However, it is expected that—given the combination of changes in precipitation variability, changed snowpack, and rising temperatures—future droughts will be more severe when they do occur. Undoubtedly, Montanans will continue to experience periodic drought, particularly in connection with climate oscillations (or equivalently, teleconnections, as described in the Climate chapter) (Trenberth et al. 2014). Thus, we discuss the implications of more severe drought, particularly during dry periods that may amplify drought effects, although we do not assume a change in frequency or duration of drought.
It is important to note that current forest conditions will largely determine the potential impacts from current and future climate change. Forest conditions vary across land ownership types, and many Montana forests are under stress due in part to past forest management practices. For example, fire suppression practices on some state and federal lands have led to denser forests, which are more susceptible to fire and stressed by competition and crowding from other trees. Further, harvest practices have shifted the genetic makeup of some forests, potentially reducing their resilience to climate change (see Genetics sidebar).
A note on species-level effects
We do not detail potential responses of individual tree species to climate shifts in this assessment; we instead direct the reader to Chapter 6 in the Northern Region Assessment Program report (Keane et al. forthcoming). That report reviews tree genetics, species distribution, potential adaptive strategies, and susceptibility to drought and disturbance from fire and insects. While the literature is inconsistent about the susceptibility of certain species to direct and indirect impacts of climate change, the relative susceptibility of tree species to drought, fire, and insect/disease has been assessed based on expert opinion (Table 4.2). Species responses will strongly depend on the magnitude of climate change, water availability, management practices, and local conditions.
Species | Drought | Fire | Insect/ Disease |
Alpine larch | Low | High | Low |
Aspen | Low-Mod | High | Moderate |
Cottonwood | Low-Mod | Moderate | Low-Mod |
Douglas-fir | Low-Mod | Low-Mod | Moderate |
Engelmann spruce | Low-Mod | Mod-High | Low-Mod |
Grand fir | Mod-High | Mod-High | Mod-High |
Limber pine | Low | Mod-High | Mod-High |
Lodgepole pine | Moderate | Moderate | Mod-High |
Ponderosa pine | Low-Mod | Low | Moderate |
Subalpine fir | Low-Mod | High | Moderate |
Western larch | Mod-High | Low | Low-Mod |
Western white pine | Moderate | Low | Mod-High |
Whitebark pine | Mod-High | Moderate | Mod-High |
DIRECT EFFECTS OF CLIMATE CHANGE ON FORESTS
Key Messages
- Increased temperatures will have positive or negative effects on individual trees and forest-wide processes, depending on local site and stand conditions. In relatively cool and moist areas, increased temperatures can improve reproduction and establishment, lengthen the growing season, and increase forest growth and productivity. Alternatively, in areas that are already warm or projected to see large temperature increases, warming is likely to decrease growth and increase heat- and drought-related mortality. [high agreement, moderate evidence]
- Direct effects of climate change on individual trees will be driven by temperature in energy-limited forests and moisture in water-limited forests. Increased temperatures and water availability could benefit forest regeneration and growth, particularly in higher-elevation forests in the northern and western parts of the state. Alternatively, decreased water availability, such as in Montana’s southeast, or south-facing slopes, will likely increase tree mortality. [high agreement, moderate evidence]
- The speed and magnitude of climate change may mean that increased forest mortality and contractions in forest distributions will outpace any gains in forest growth and productivity over the long run, leading to a net loss of forested area in Montana. However, range shift responses will be highly dependent on species and region. [medium agreement, limited evidence]
The direct effects of climate change on forests include increased temperatures and shifts in precipitation that together can alter humidity, soil moisture, and water stress. These effects result in short-term and long-term impacts to tree establishment, growth and productivity, and mortality. In addition, elevated CO2 levels may influence forest growth, productivity, and water use.
Direct effects can be beneficial or detrimental to forest growth and survival. Each tree species will respond differently to climate variation depending on its specific physiological tolerances. Direct effects overlie existing forest conditions arising from past and future human land-use activities (Moritz and Agudo 2013). Thus, net impacts, whether positive or negative, may be difficult to estimate and will vary substantially across Montana.
Forest patterns and conditions depend on the life cycles of individual trees and forest-wide processes. In the remainder of this section, we review how different aspects of climate may influence three primary life-cycle stages or forest processes: seedling establishment and forest regeneration; tree growth and forest productivity; and tree mortality and forest die-off (findings summarized in Table 4-3). Life-cycle stages and forest processes may occur at different temporal and spatial scales, but are related and we discuss each in turn. We close the section with a discussion of changes in species distribution that might be expected from the direct effects of climate change.
Direct effect | Possible impacts | Projected net effect |
Establishment and regeneration |
Positive: Higher CO2 concentrations and temperatures may lead to increased tree fecundity Negative: Higher temperatures and reduced water availability could reduce seedling survival |
Possible positive or negative effects are superimposed on climate oscillations, such as the Pacific Decadal Oscillation, which can produce decades of cooler and wetter conditions that may be more favorable for establishment and regeneration |
Growth and productivity |
Positive: Increased vegetation water use and increased growth and productivity as a result of longer growing season Negative: Reduced growth and productivity in water limited areas |
Possible increased growth and productivity concurrent with climate oscillations that increase water availability, particularly at higher elevations and where stand density is low; extreme high temperatures would have net negative impact, regardless of water availability |
Mortality |
Positive: Few opportunities for reduced direct climate effects on mortality but possibility for reduced mortality from indirect effects Negative: Increased acute and background mortality from increased temperatures and indirectly from increased disturbance |
Increased mortality, although may be driven by indirect effects; patterns of mortality will be dependent on initial stand and local site conditions, but more arid regions more susceptible |
Range shifts and forest distribution |
Positive: Potential range expansion with warmer temperatures and sufficient moisture Negative: Potential range contraction where temperature is too high or in water-limited locations |
Possible faster range contraction compared to expansion, with net range reduction particularly in drier areas; no clear direction of elevational shifts; responses will be highly species and location dependent |
Establishment and regeneration
Although tree recruitment—the process of a seedling becoming established and surviving into adulthood—is affected by many factors, the process is strongly tied to temperature and water availability (Ibáñez et al. 2007). Overall, studies are inconclusive as to net impacts of changing atmospheric chemistry and climate on seedling establishment and growth. Given the climate projections of increased winter and spring precipitation, but drier summers, predicted uncertainties of seedling regeneration are uncertain.
Climate conditions determine the window of time for successful seedling establishment (Ibáñez et al. 2007). Warmer conditions combined with wetter winters and springs may lengthen the window for seedling establishment in high-elevation forests, but may not change significantly in lower elevation forests (though it may shift earlier) (Keane et al. forthcoming). However, even with a lengthened window for establishment, warming temperatures alone may cause seedling mortality and failed regeneration as a result of seasonal mismatches in the timing of flowering and seed production (Cayan et al. 2010; Williams et al. 2013). In the short term, warmer temperatures but drier summers may increase forest regeneration due to a) increased tree flowering and recruitment, and b) drought-related reductions in canopy cover speeding sapling growth (Galiano et al. 2013; Ibáñez and McCarthy-Neumann 2014; Clark et al. 2016). Additionally, higher CO2 levels have been shown to increase fertility and seed and pollen production in some trees (Ladeau and Clark 2006). However, in the long term, more severe drought is likely to reduce tree establishment by reducing seed germination, as well as increasing mortality of seedlings and saplings (Kolb and Robberecht 1996; Chmura et al. 2011).
Many responses may be species-specific, for example ponderosa pine seedlings are sensitive to temperature, lodgepole pine seedlings are sensitive to moisture fluctuations (Petrie et al. 2016). Ultimately, in forests not otherwise limited by energy or nutrients variability in moisture availability with natural and climate oscillations may drive establishment success between years (League and Veblen 2006), with indirect disturbance effects (e.g., fires, landslides, insect outbreaks, and pathogen attacks) greatly affecting long-term recruitment success (Clark et al. 2016).
Growth and productivity
Warming temperatures, increased atmospheric CO2, and longer growing seasons provide opportunities for increased photosynthesis, thereby improving forest growth and productivity (Ehleringer and Cerling 1995; Joyce and Birdsey 1995; Waring and Running 2007; NPS 2010). However, these same changes can also reduce forest productivity, particularly in water-limited systems. Thus, net forest response is uncertain, but likely negative under extreme temperature increases.
Forest productivity will increase up to some optimal temperature and then begin to decline if temperatures continue to rise. This decrease results because plant respiration also increases with temperature, and some of the photosynthetic gains (that lead to increased productivity) are lost through a) growth and maintenance respiration (Ryan et al. 1995), or b) seasonal differences between photosynthetic gains in the spring and increased respiration in the fall. These tradeoffs can result in no net increase in productivity (Piao et al. 2008). Additionally, extremely high temperatures can lead directly to increased water stress because of drier soils. The temperature threshold at which declines would occur is complicated by the fact that elevated CO2 levels may increase water-use efficiency in plants (Waring and Running 2007) and thereby lower plant water stress (Franks et al. 2013).
Although CO2 fertilization has likely increased forest growth at a global scale (McMahon et al. 2010), this increase may be evident in only about 20% of forests, with the remaining 80% unable to capitalize on benefits of higher atmospheric CO2 because of water or nutrient limitations (Gedalof and Berg 2010). A recent study suggests that Montana forests will likely show substantially lower productivity overall given only small projected increases in precipitation (Charney et al. 2016). Reduced snowpack and earlier snowmelt (see Water chapter) may further limit any potential gains in productivity. In general, changes in temperature, precipitation, and snow could alter forest productivity in Montana as described below.
- Forest productivity may increase in montane, subalpine, and alpine areas.—These areas may not exceed optimal temperatures, even under end-of-century projections, and these high-elevation areas should have sufficient moisture with increased winter and spring precipitation and longer snowpack duration, allowing growing seasons to lengthen and forests to benefit from higher atmospheric CO2 (Keane et al. forthcoming). Gains in forest productivity have already been observed in relatively cooler/wetter sites at higher elevations and northern range limits in other regions (Littell et al. 2008; Bhuta et al. 2009; Salzer et al. 2009; D’Orangeville et al. 2016).
- Forest productivity may decrease in lower elevation, warmer, and drier sites.—Conversely, lower elevation areas are likely to see more extreme high temperatures combined with low soil water availability later in the year, resulting in reduced productivity.
Ultimately, shifts in productivity will be site- and species-specific and vary across years. Above- and below-normal temperature and precipitation years associated with natural climate oscillations may determine whether growing seasons lengthen, contract, or shift in time. But even under ideal temperature and moisture conditions, productivity gains will be dependent on local site conditions, such as where there are sufficient soil nutrients or where stand density is low and little competition exists for available resources (Ford et al. 2016). Further, if extreme heat events increase substantially, impacts will be negative regardless of water availability.
Mortality and die-off
The expected increase in drought severity will increase tree mortality in forests. Already, widespread, catastrophic forest die-off events throughout the western US have been directly or indirectly related to drought (Breshears et al. 2005; Allen et al. 2010; Ganey and Vojta 2011; Worrall et al. 2013). Multiple researchers have shown that extended drought correlates with declining tree growth and increased risk of mortality (Allen et al. 2010; O’Connor 2013; Williams et al. 2013). Similarly, the combination of increased warming and drought conditions is the likely cause of recent rapid increases in background (non-catastrophic) forest mortality rates in Montana and the interior West (van Mantgem et al. 2009). For trees beyond the seedling stage, increased temperatures may be responsible for tree mortality more so than water stress (Luo and Chen 2013), although water stress may have been more important historically (Rapacciuolo et al. 2014).
Initial forest conditions affect levels of tree mortality from direct climate effects. For example, soil type and depth, elevation, and aspect all influence water availability for forests. Stand condition may also increase tree mortality by increasing the likelihood of indirect effects (discussed below). Stand condition is particularly important on state and federal forests where a policy of fire suppression for the last 100 yr has increased tree density and the risk of mortality from defoliating and boring insects, and from wildfire.
Species range shifts and forest distribution
Climate conditions and disturbance regimes largely control plant distributions (ranges). Over the millennia, the main responses of species to climate change has been to adapt to changing conditions, move to a new site (range shift), or go extinct (Davis and Shaw 2001). Although many tree species in Montana are relatively plastic—meaning they can adapt in the short term to a wide range of climate conditions—this plasticity could be challenged by severe or prolonged drought or substantially modified disturbance regimes (Allen et al. 2010). Long-term adaptability is determined by the genetic diversity of forests and individual species (Davis et al. 2005), both of which may have been reduced by lack of understanding in the past of how forest management activities affect forest genetics (see Genetics sidebar).
The Importance of Genetic Diversity Forest genetics—the genetic variation and inheritance of various genes of forest trees—will primarily determine a forest’s ability to adapt to climate change over the long term. Genetic diversity largely determines a species’ ability to survive extreme events and adapt to changing conditions (Ledig and Kitzmiller 1992). Historically, the high genetic diversity of many tree species allowed forests to tolerate a wide range of environmental conditions and adapt to shifts in climate (Westfall and Millar 2004; Nicotra et al. 2015). However, it is unclear whether species with even high levels of genetic and physical diversity can adapt fast enough to the rapid and extreme shifts in climate that are projected over the next century (Vose et al. 2012). Human actions have undoubtedly altered forest genetics, at least in part through silvicultural practices. Because physical characteristics (e.g., tree height or basal diameter) are used to select trees for harvest, silvicultural practices can alter forest genetics, which are the bases for these physical differences. Selective harvesting may have substantially altered the presence of rare genetic characteristics (Cheliak et al. 1988; Schaberg et al. 2008), which are often those needed by a species to adapt to climate change. Even though data regarding trends in forest genetic diversity are scarce, a general consensus exists that natural genetic diversity may be at risk globally as a result of human activities (Schueler et al. 2012; Alfaro et al. 2014; FAO 2014). Managing forests to retain or increase this diversity is one of the best options for conservation. |
||
Some evidence exists that tree ranges are already shifting to colder locations in the Pacific Northwest (Monleon and Lintz 2015). However, climatically suitable places are often geographically limited, and alpine vegetation may be running out of mountain as it seeks colder climes (Gottfried et al. 2012). To complicate matters, the optimum elevations for some plant species are shifting downhill tracking changes in water availability, as opposed to simply moving uphill, tracking changes in temperature (Crimmins et al. 2011). Divergence in the direction between optimal temperature and moisture conditions may make it difficult for trees to stay in equilibrium with favorable climate conditions (Dobrowski et al. 2013). Regardless of direction of range shift, there is concern whether tree species can disperse and regenerate quickly enough to keep pace with the magnitude and rate of projected climate changes (Zhu et al. 2012). Although dynamic vegetation models tend to predict an overall expansion of cool forests and woodlands (Shafer et al. 2015), some tree species may actually experience reduced ranges due to geographical obstacles to range expansion in response to climate (Coops and Waring 2001). Current best global estimates suggest that forest mortality is outpacing benefits from increased tree productivity due to increased atmospheric CO2 (Allen et al. 2010), signifying an overarching contraction of forest range (Dobrowski et al. 2015).
Feedbacks Forest responses to climate change may be complex due to feedbacks, which are the interplay between different climate change-related effects. One effect may amplify or diminish another effect, and that interaction has the potential to result in changes that are non-linear, unpredictable, and even dramatic. For forests, a notable challenge will be understanding and preparing for interactions in disturbances (Buma 2015). For example, if a fire burns a forest, it will release stored carbon. If many fires burn globally, there will be an increase in atmospheric CO2, the main driver of climate change. Further, more fires would mean fewer trees and that could mean that less water vapor transpired into the air, resulting in drier conditions. Drier and warmer conditions would lead to more fires—a positive feedback loop where changes are amplified. In a negative feedback loop, changes are diminished or reach a steady state. For example, increased atmospheric CO2 may increase forest productivity. More trees would sequester more atmospheric CO2, thereby reducing atmospheric CO2 and dampening the initial CO2 fertilization effect. A simplified example of the feedbacks between wildfire and climate (adapted from Vose et al. 2016).
Feedbacks may occur between resources as well, for example, between forests and water quantity. The feedbacks are not always as assumed. For example, forest die-off caused by drought has, in some areas, been shown to reduce streamflow as opposed to increasing it, as might seem more intuitive (Guardiola-Claramonte et al. 2011). Even where ecologists recognize a feedback, they often do not understand all the connections or even if the interplay between components leads to positive or negative outcomes. It is also likely that many feedbacks exist that are currently unknown. Regardless, scientists believe that feedbacks are likely to increase the ecosystem impact of individual disturbances, which may have substantial implications for changes in distribution and heterogeneity of forests in the future (Bonan 2008; Vose et al. 2012; Richardson et al. 2013). |
||
INDIRECT EFFECTS OF CLIMATE CHANGE ON FORESTS
Key Messages
• An increase in fire risk (i.e., probability of occurrence)—including an increase in size and possible frequency and/or severity (i.e., tree mortality)—is expected in the coming century as a result of a) prolonged fire seasons due to increased temperatures, and b) increased fuel loads from past fire suppression. Spatial patterns of fire activity will be complex and dependent on disturbance history and current stand condition. Fire risk may increase in all forests; fire severity may increase the most in lower elevation forests. [high agreement, robust evidence]
• Rising temperatures are likely to increase bark beetle survival [high agreement, strong evidence], but climate-induced changes to other insects and forest pathogens are more varied and less certain [medium agreement, moderate evidence]. Climate change effects are difficult to forecast because of the interplay between climate-driven changes in insect or pathogen behavior and changes in host tree susceptibility.
• There may be a reduction in the amount of carbon stored in forests. Rising temperatures and increased atmospheric CO2 can increase forest productivity and thus the carbon stored in organic matter. However, warmer temperatures can also reduce soil carbon through increased decomposition rates. Overall, increased tree mortality from increased forest disturbance may cause a reduction in forest carbon storage. [low agreement, limited evidence]
The direct effects of increasing temperature and precipitation may result in the expansion and/or contraction of certain forest types in certain regions of Montana. However, the indirect effects of climate change on forests, such as changing wildfire and beetle outbreak severity, are already having a large impact on the health of Montana’s forests and in some instances these impacts are easier to predict. These direct and indirect impacts of climate on forests may be exacerbated or ameliorated by human land-use activities in the past and moving forward.
Our scientific understanding of disturbance associated with extreme weather events limits our ability to project landslides, blow downs, ice storms, and other such events in the future. In this section, we will consider the impact of changes in fire, insect, and pathogen outbreaks on forests, as well as on soil and carbon storage, for which we have better capacity for forecasting (Table 4-4).
Indirect effect | Possible impacts | Projected net effect |
Disturbance: fire |
Positive: Increased forest heterogeneity (long-term, post-burn) Negative: Decreased forest diversity and heterogeneity (immediately post-burn); increased social and economic impacts from fire; increased release of forest carbon |
Increased fire severity resulting primarily from warmer weather and past fire suppression; increased release of forest carbon from fire |
Disturbance: pathogens |
Positive: Some pathogen species may decline and result in decreased forest mortality Negative: Some pathogens species may increase and result in increased forest mortality and increased susceptibility to beetle attack |
Uncertain climate effects on pathogens, dependent on moisture regimes, pathogen species, and host species |
Disturbance: insects |
Negative: Increased forest mortality; reduced forest diversity with shift towards non-host tree species |
Increased temperatures likely to result in increased insect disturbance, but dependent on elevation, insect species and host availability |
Soil responses and carbon storage |
Positive: Increased organic matter if increased productivity; increased nitrogen availability Negative: Decreased organic matter (with increased decomposition rates); decreased mycorrhizal support; increased soil acidity; increased release, or decreased removal, of atmospheric CO2 |
Uncertain climate effects on soil responses, but projected reductions in soil and forest carbon storage |
Disturbance resulting from fire
Fire is a critical component of forest dynamics; it has historically been the dominant disturbance in forest ecosystems in the West (Baker 2009; Marlon et al. 2012). Fire regimes are characterized by interactive relationships, across temporal and spatial scales, between climate and weather, vegetation and fuels, and ignition sources and topography (Parisien and Moritz 2009). Fires have burned frequently and widely across Montana (Figure 4-4), but the area burned and the severity of fire, often measured by reduction in biomass or tree mortality, has varied across the state. For example, from 2003-2012, fire severity was greatest along the eastern border and southern portion of the northwestern climate division (Figure 4-5) (Berner et al. forthcoming).
Historical and Recent Fires in Montana |
![]() |
Figure 4-4. Extent and location of historical and recent fires in Montana, 1889-2013. Historical data (1889-1991) are mapped as actual fire boundary polygons as available. Recent data (1992-2013) are mapped as circles approximating burned area. Recent fires too small to be seen by area are mapped as points. Forests are shown in green. Fire data represent primarily forest fires, but may include grassland and other fire types. Brown boundaries delineate climate division. Data and map from Hoff (forthcoming). |
Fire Severity |
![]() |
Figure 4-5. Fire severity (measured as total carbon stored in aboveground tissues killed by fire) estimated for 2003-2012, a relatively dry decade. Adapted from Berner et al. (forthcoming). |
Broadly, seasonal average temperature and precipitation patterns limit both the length of the fire season and environmental conditions during the fire season. Over the long term, climate also determines the distribution of vegetation and possible fuels (Power et al. 2007; Marlon et al. 2008).
Fire occurrence increases during hotter, drier conditions (called fire weather) (Flannigan and Harrington 1988). Thus, the fire season in Montana typically extends from late June through October at lower elevations, with shorter seasons at higher elevations where snowpack can persist into July (Keane et al. forthcoming). Variation in fire regimes in the West have historically been associated with climate oscillations (Heyerdahl et al. 2002). In Montana, increased fire frequency is associated with warmer spring temperatures and drier summer conditions (Heyerdahl et al. 2008; Morgan et al. 2008), often associated with El Niño. The phase of the Pacific Decadal Oscillation that leads to warmer conditions may also prolong and intensify the fire season (Heyerdahl et al. 2008; Jolly et al. 2015; Abatzoglou and Williams 2016), and it is clear that years with protracted or widespread wildland fire or increased fire severity are correlated with drought (Littell et al. 2009; van Mantgem et al. 2013). Warmer, drier climate phases can particularly increase fire risk when they follow cooler, wetter conditions that increase fire fuel availability via increased vegetation growth and reduced fire activity (Heyerdahl et al. 2008). Topography can also influence fire behavior by determining local microclimates—for example, variations in local snowpack, temperature, and humidity (Holden and Jolly 2011)—or alignment with prevailing winds (Sharples 2009) which increase fire spread.
Fires in Montana, 1970-2015 |
|
Figure 4-6. Number of fires in Montana, 1970-2015, by month of occurrence (NIFC undated). |
Across Montana, conditions that lead to high fire risk (i.e., likelihood of occurrence) are becoming more common: seasonal maximum temperatures are increasing, snowmelt is occurring earlier, minimum relative humidities are decreasing, and fuels are becoming drier (Jolly et al. 2015; Seager et al. 2015). Combined, these factors have led to the fire season lengthening globally between 1979 and 2013 (Jolly et al. 2015). In addition, across the western US, fuel loads and tree densities have increased as a result of fire suppression practices beginning in the 1920s (Parks et al. 2015), as well as other land uses, such as timber harvest and grazing (Allen et al. 2002; Swetnam and Betancourt 2010). As a result, it is clear that climate change, combined with greater fuel loads, has increased western fire activity over the past 30 yr (Westerling et al. 2006; Miller et al. 2008; Dennison et al. 2014; Abatzoglou and Williams 2016).
Combined with fuel loads, higher evapotranspiration rates and resulting shifts in water balance may be the best predictor of increased fire risk and fire severity in the future under a changing climate (Littell and Gwozdz 2011; Abatzoglou and Kolden 2013). Yet, climate change effects on overall water balance are uncertain. Rising temperatures should increase evapotranspiration, but plants may adapt by reducing water lost to transpiration. Additionally, vegetation patterns and forest connectivity, and the feedback between these and fire, play an important role in how climate-driven changes in fire regimes are likely to play out over the long term (McKenzie and Littell 2017).
Although fire modeling is complex and models specific to Montana climate divisions are unavailable, recent studies suggest likely trends for the state. McKenzie and Littell (2017) project that water balance deficits will increase, likely leading to increased area burned. Jolly et al. (2015) project that warmer summers and reduced moisture will also continue to lengthen fire seasons. Modeling work by Schoennagel et al (2004) and Rocca et al (2014) for the Rocky Mountains projects changes in fire frequency (assumed by the authors to be related to the long-term increase in probability of fire occurrence) and severity in western Montana. Those changes, for broad forest-type categories over the short and long terms, are shown in Table 4-5. These projections likely are generally applicable to other parts of Montana, as well.
Forest type | Short-term impact | Long-term impact |
In lower montane forests, primarily consisting of ponderosa pine, co-dominated by Douglas-fir or western larch | Increased fire weather could lead to short term increased fire frequency and fire severity, particularly where fire suppression efforts have increased fuel loads | Reduced fire frequency and increased fire severity in the long-term. |
In cooler and wetter upper montane forests | Increased fire frequency and severity | Increased fire frequency but uncertain implications for changes in fire severity |
Subalpine forests, dominated by Engelmann spruce and subalpine fir species or by lodgepole pine mixed with limber pine or whitebark pine in drier sites | Increased fire frequency with no change in fire severity | Increased fire frequency with no change in fire severity |
Disturbance resulting from pathogens and insects
Dale et al. (2001) estimated that impacts from forest pathogens and insects result in greater economic costs to US forests than any other type of disturbance. Pathogens include fungal, bacterial, and viral infections, as well as parasitic plants (and here we include general forest disease in our definition of forest pathology). Pathogens can affect different parts of a tree, such as trunk and branch cankers, root pathogens, and foliar (leaf or needle) diseases.
Many of these insects and pathogen species are native to Montana’s forests. For example, mountain pine beetle (Dendroctonus ponderosae), Douglas-fir beetle (Dendroctonus pseudotsugae), and the western spruce budworm (Choristoneura occidentalis; a defoliator) are important in determining forest distribution, structure, and regeneration. Although these native insects are unlikely to annihilate their host species, recent extreme outbreaks have severely impacted some western forests. Non-native species, conversely, have the ability to eradicate their host species locally. For example, white pine blister rust, caused by the fungus (Cronartium ribicola), has put western white pine, limber pine, and whitebark pine in some areas of Montana in jeopardy (Smith et al. 2008).
Using aircraft, the US Forest Service has conducted insect and disease detection surveys for over 50 yr (USFS 2016). Nearly 14 million forested acres (5.7 million ha) in Montana showed visual signs of disease or insect disturbance between 2000-2015 (Figure 4-7), and that number is assumed to be conservative. Bark beetles and defoliators have been the primary cause of biotic disturbance as identified from aerial surveys (Figure 4-8). Based on other, non-visual, approaches to estimate risk from pathogens and insects, root disease appears to be another major threat to Montana’s forests (Krist et al. 2014).
Climate change can influence forest pathogens and insects through three primary mechanisms: 1) altering pathogen or insect abundance and distribution via physiological effects; 2) altering tree defenses; and 3) altering interactions between pathogens, insects, and their competitors (Weed et al. 2013). In addition, climate change can alter the distribution and presence of host species.
The interplay between these mechanisms complicates efforts to forecast the potential effects of climate change on pathogens and insects. Soil water deficits, from increased temperatures and reduced precipitation, can result in larger pathogen populations and lower tree and forest defenses against pathogens (Lorio 1993; Chakraborty et al. 2008). Many pathogens tolerate greater water stress than the trees they infect, and some fungi that commonly occur in or on trees become pathogenic when a tree is water stressed (Desprez-Loustau et al. 2006). Beetle activity is also strongly tied to climate, and warmer temperatures speed up reproduction times, extend growth periods, and increase probability of beetle survival (Mitton and Ferrenberg 2012; Bentz and Jönsson 2015; Bentz et al. 2016). Further, trees stressed by drought are more susceptible to beetle invasions (Creeden et al. 2014). Field studies suggest that recent mountain pine beetle outbreaks correlate with mean August temperatures >59°F (15°C) and that outbreak size is correlated with minimum winter temperatures and drought conditions in previous years (Preisler et al. 2012).
Forest Disturbance |
![]() |
Figure 4-7. Recent Montana forest disturbance as visually estimated from aerial surveys in 2000-2015 (USFS 2016). Forests are shown in green. Darker gray background represents area surveyed in 2015; not all areas were surveyed in all years and many pathogens cannot be visually estimated. Brown boundaries delineate climate divisions. |
Forest Disturbance |
![]() |
Figure 4-8. Forest disturbance in Montana from 2000-2015 by type of visually surveyed pathogen or insect as percentage of the total area surveyed from USFS (2016) Aerial Detection Survey data. |
Projections of continued forest mortality from pathogens and insects suggest that substantial portions of western Montana are at high risk regardless of climate change (Figure 4-9) (Krist et al. 2014). Krist et al. (2014) suggest that expected climate changes would increase pathogen and insect risks to forests beyond those mapped in Figure 4-9 or, at a minimum, alter the spatial patterns of risk.
Forested Areas at High Mortality Risk |
![]() |
Figure 4-9. Forested areas (green) at high risk of mortality (red) from combined insect and pathogen attacks from the National Insect and Disease Risk Map (Krist et al. 2014). This map does not consider increased risks from projected climate changes. Areas in red are locations where it is estimated that 25% or more of live trees with a diameter of greater than 1 inch (2.5 cm) are at risk of mortality by 2027 from insects and disease. |
Changes in pathogen activity may be most strongly linked to shifts in precipitation patterns and moisture availability. For example, Sturrock et al. (2011) estimate that a) Dothistroma needle blight (Dothistroma septosporum or D. pini), whose primary host in Montana is ponderosa pine, will have reduced or increased impacts, depending on warmer and drier or wetter conditions, respectively; and b) Armillaria root disease (Armillaria spp.), which generally affects Douglas-fir and grand fir, will have increased impacts under warmer, drier conditions, but no change under warmer, wetter conditions. Sturrock et al. (2011) also state that the implications of climate change for white pine blister rust are uncertain, but suggest decreased impacts under a warmer, drier climate, and no change under warmer, wetter conditions because infections require both moist and cool environments.
It appears more certain that a warming climate will increase insect-related forest mortality, depending on the presence of susceptible host trees. Already, warming temperatures have expanded the range of beetles (Carroll et al. 2006), and the largest recorded bark beetle epidemic in western forests has occurred in the past 15 yr. Higher temperatures, if large enough, lead to more severe droughts as water is more rapidly and completely evaporated from soils and streams, which may in turn make forests more susceptible to western spruce budworm outbreaks (Régnière et al. 2010; Flower et al. 2014).
Future of the Mountain Pine Beetle in Montana The mountain pine beetle (Dendroctonus ponderosae) epidemic is currently on the wane in Montana because of the reduction of susceptible host trees. We project, however, that rising winter temperatures will result in increased mountain pine beetle populations. Those increases will result from fewer cold snaps, and hence substantially decreased likelihood of seasonal mountain pine beetle die-off. We came to that conclusion using field data from Idaho (Régnière and Bentz 2007)—to assess threshold cold temperatures by month (beetles adapt to colder temperature as the winter progresses) that cause approximately 50% mortality in mountain pine beetle populations—combined with projected future air temperatures. For air temperatures, we used four climate scenarios, as described in the Climate chapter, and projected the number of days that are likely to exceed those threshold winter temperatures in the future. Higher winter temperatures, then, equate to reduced winter die-off (i.e., larger populations) of mountain pine beetles. The figure below shows that for all four temperature scenarios studied in this assessment, warm winter days, above the temperature threshold necessary to kill about half of the mountain pine beetles, will occur more frequently. In other words, warming winter temperatures are projected to increase mountain pine beetle survival. Our assessment did not show substantial differences across elevations (we considered low, moderate, and high elevations for known pine locations), but other studies have (Hicke et al. 2006; Raffa et al. 2013; Bentz et al. 2016). All insects have different survival strategies and climate tolerances, and even the mountain pine beetle, about which much is known, has a very complex life history (Bentz and Mullins 1999). Thus, impacts could be greater than would be indicated by only considering warmer winter temperatures (Buotte et al. 2016). However, the mountain pine beetle provides a good example of how potential changes in temperature may impact insect species that disturb Montana forests.
(left) Mountain pine beetle (Dendroctonus ponderosae). (above) Percent increase in number of days per month exceeding the threshold cold temperatures necessary to cause approximately 50% mortality in mountain pine beetle populations as projected under two greenhouse gas emission scenarios (i.e., representative concentration pathways; see Climate chapter) at mid century and end-of-century. |
||
Soil responses, nutrient cycling, and carbon storage
Soils that are high in organic matter support forest resources by providing moisture, necessary nutrients, and physical support; filtering of toxics and other unwanted compounds; and helpful biota such as mycorrhizae, which are a symbiotic relationship between fungi and roots that help a tree absorb nutrients and water. Soils high in organic matter also store more carbon. Climate change can affect all of these soil functions.22
Changes in soil temperature and moisture can have substantial impacts to forests, although the direction of change and resulting impacts are uncertain. For example:
- Soil decomposition rates may increase with high temperatures, reducing the quality and quantity of soil organic matter (Keane et al. forthcoming). Similarly, wet soils can increase decomposition rates, but it is unknown whether soil moisture will increase or decrease under projected climate changes for Montana. Alternatively, increases in forest productivity resulting from increased atmospheric CO2 can increase litter and soil organic matter.
- Nutrient cycling may be affected by rising temperatures that, in turn, can increase microbial activity. This feedback has the potential to increase nitrogen deposition, providing more of a nutrient critical to tree growth (Melillo et al. 2011). Warming temperatures can also increase nitrogen export (reduction). Recent work by Brookshire et al. (2011) suggests that climate change-driven loss of soil nitrogen could outpace deposition by 3 to 1.
- More important may be how multiple, climate-related effects interact to impact soil resources. For example, increased disturbance from fire or insects could reduce forest canopy shading, thereby further compounding the effects of rising air temperature on soil temperatures. Increased temperatures and reduced water, happening concurrently, can decrease mycorrhizal colonization of tree roots (Compant et al. 2010), exacerbating a tree’s susceptibility to pathogens.
- Carbon storage may also be impacted by climate change. At a global scale, almost 45% of the total forest storage of carbon is in soils, with most of the rest (approximately 42%) stored in live woody biomass (Bonan 2008; Pan et al. 2011). Global forest carbon storage has been increasing over the past 50 yr in response to increased nitrogen deposition and atmospheric CO2 concentrations, despite a worldwide reduction in forested area (Bellassen and Luyssaert 2014). The same trend is seen in the USFS’s Northern Region (northeastern Washington, northern Idaho, and Montana) between 1990-2013, although the trend is small and there are substantial differences among forest types (USFS 2015).
Researchers debate whether increasing temperatures will increase or decrease carbon storage (Davidson and Janssens 2006). With rising temperatures, carbon storage will increase due to increasing forest productivity (assuming adequate water and nutrient availability) (Finzi et al. 2006; Norby et al. 2010; Garten et al. 2011), but decrease due to increasing microbial respiration, which in turn releases CO2 from the soil. Changes in disturbance regimes (e.g., increased fire frequency or tree mortality from insects) could also result in release of carbon currently stored in forests (Baldocchi 2008; Kurz et al. 2008; Loehman et al. 2014). Overall, carbon models suggest that increased fire and bark beetle outbreaks are likely to reduce carbon storage in western forests (Metsaranta et al. 2010; Westerling et al. 2011), and some research suggests recent steep declines in forest carbon storage in the Rocky Mountains as a result of higher rates of disturbance relative to historical values (Wear and Coulston 2015).
ADAPTATION STRATEGIES FOR A CHANGING CLIMATE
Managers should consider multiple scenarios of potential climate shifts and contemplate a suite of adaptation strategies. Vose et al. (2012) suggest four general types of adaptation options in managing forests for the potential impacts of climate change:
- Promote resistance.—Enhance ability of species of system to resist forces of climate change;
- Increase resilience.—Enhance capacity of system to absorb impact without substantial changes to processes and functionality;
- Enable ecosystems to respond.—Assist a system’s transition to an altered state that is adaptive to a changed climate while minimizing disruptive outcomes; and
- Re-align highly altered ecosystems.—Use restoration techniques to allow a system’s function to continue through changing climate conditions.
We recommend a bet-hedging approach, understanding the range of potential options and their possible consequences, and selecting among those that provide the most likely benefit given future uncertainty. We list many potential specific adaptation strategies in Table 4-6. Several guidebooks exist for developing adaptation options on forested lands; these include Responding to Climate Change on National Forests (Peterson et al. 2011), Climate Change in Forests of the Future (Millar et al. 2007), and Forest Adaptation Resources (Swanston et al. 2016).
Table 2-3. Decadal rate of change for annual average temperatures in °F (°C) for the seven Montana climate divisions (Figure 2-3), statewide, and US from 1950-2015. A value of 0 indicates no statistically significant change between decadal averages.
Plant from multiple species, seed sources, and climate zones, particularly from locally-adapted sources Manage to maintain genetic diversity and phenotypic plasticity Create opportunities for rapid natural selection for species with high predicted potential for adverse impacts from climate change (Sturrock et al. 2011; Erickson et al. 2012; Alfaro et al. 2014; FAO 2014) Plant drought tolerant and native species Retain diversity of species and promote legacy trees Manage or restore mosaic (variable pattern of species and ages) and maintain or improve landscape connectivity Plant in asynchronous rotations and manage for diverse age classes Thin, plant, and use prescribed fire to favor species adapted to disturbance (Millar et al. 2007; Vose et al. 2016; Keane et al. forthcoming)
Adaptation option | Time period | Examples (further reading) |
Increase genetic and phenotypic diversity | Mid to long term | Breed for climate resilience and disease resistance |
Improve forest structure, diversity, and resilience | Long term | Plant various species in microsites (small areas with locally variable climate, topographical, and soil conditions) with existing species mix as guide |
Improve establishment | Short to long term | Plant drought-tolerant species in years with strong El Niño forecasts, particularly during Pacific Decadal Oscillation warm phase; plant trees that require sufficient water during establishment in La Niña years and during Pacific Decadal Oscillation cool phases Focus planting more in spring as fall planting becomes more difficult with reduced soil moisture and test different planting timings as springs shift earlier |
Improve water availability | Long term | In snow-dominant locations, reduce canopy cover on north slopes (reduce interception of moisture by canopy), retain canopy cover on south slopes (increase shading), in all locations maintain sufficient shading on south slopes to retain soil moisture |
Improve soil quality | Mid to long term | Alter timing of logging to reduce soil compaction Retain woody debris to retain soil moisture and promote nutrient cycling |
Reduce fire risk and fire severity | Mid to long term | Apply additional efforts for fire prevention in drier, warmer years; allow spot burning in cooler, wetter years Use prescribed fire and thinning to minimize fuel loading and favor fire-resistant species (Vose et al. 2016; Keane et al. forthcoming) |
Manage forest diseases | Mid to long term | Monitor, forecast, and plan regarding forest diseases and use an adaptive management framework Ensure management activities do not spread disease Breed for increased disease resistance (Sturrock et al. 2011) |
Consider assisted migration and adaptation | Mid to long term | Assisted adaptation—often defined as management to assist gene flow or selection for specific genetic traits—may be a more useful tool than assisted migration whereby a species is deliberately moved to a different habitat; carefully consider implications of either action Identify potential climate refugia to focus restoration efforts Plant a mix of seeds genetically selected and adapted to likely future and current conditions (McLachlan et al. 2007; McKenney et al. 2009; Aitken and Whitlock 2013; Alfaro et al. 2014) |
Manage forest carbon (mitigation) | Mid to long term | Planting new trees to increase forested area Increase carbon storage in existing forests (e.g., replace dying stands, manage for maximum productivity and reduced fire risk via pre-commercial thinning23) Use of wood as biomass energy (McKinley et al. 2011; Bellassen and Luyssaert 2014) |
KEY KNOWLEDGE GAPS
Many knowledge gaps still need to be filled to better understand and prepare Montana’s forests to survive and thrive under a changing climate. We detail 12 key needs below to achieve better understanding of direct effects, indirect effects, and general effects.
Better understanding of direct climate effects.—1) Improved understanding of adaptive genetic and phenotypic forest characteristics that would provide better guidance for breeding programs and management actions to maximize resilience to both direct and indirect climate impacts to forests; 2) Long-term studies to better understand effects of CO2 fertilization in Montana’s forests; 3) Improved models of climate and vegetation effects on evapotranspiration and water balances throughout forested systems.
Better understanding of indirect climate effects.—4) Improved fire models and projections directly related to Montana’s forests; 5) Long-term monitoring of forest insect and pathogen response to recent climate changes and improved projections of likely future impacts; 6) Better understanding of disturbance effects on microclimates and refugia and implications for forest productivity, mortality, and adaptation.
Better understanding of general effects and adaptation options.—7) Forest models for Montana that account for changes in both climate and resulting vegetation distribution and patterns; 8) Models that account for interactions and feedbacks in climate-related impacts to forests (e.g., changes in mortality from both direct increases in warming and increased fire risk as a result of warming); 9) Systems thinking and modeling regarding climate effects on understory vegetation and interactions with forest trees; 10) Discussion of climate effects on urban forests and impacts to cityscapes and livability; 11) Monitoring and time-series data to inform adaptive management efforts (i.e., to determine outcome of a management action and, based on that outcome, chart future course of action); 12) Detailed decision support systems to provide guidance for managing for adaptation.
CONCLUSIONS
Much is known regarding how forest ecosystems will respond to climate change, even amid the uncertainties. Two conclusions can be made with high confidence:
Rising temperatures and shifts in precipitation and moisture balance of forests are likely to increase negative direct effects on forests, particularly in water-limited systems and in years with low precipitation.
In some regions, indirect effects of climate, due primarily to increased frequency and severity of wildfire and beetle outbreaks, will have a greater impact than direct climate effects.
By instituting adaptation and mitigation programs, forest managers can act now to lessen the likelihood and magnitude of climate change impacts on Montana’s forests. Such programs, best undertaken in an adaptive management framework, include, but are not limited to, reducing fire risk; managing forest diseases; improving forest establishment; increasing forest carbon storage; improving water availability and soil quality; improving forest diversity, structure, and resilience; and increasing genetic and phenotypic diversity of forests.
|
Socio-cultural ConcernsSacred sites and traditional first foods.—Foods play a vital role in physical, mental, and spiritual health of indigenous communities. Many tribal communities rely on first foods for sustenance, and these foods are equally important to the sustenance of tribal culture. In Montana, examples of first foods and plants that are found in forests and open woodlands include camas (Camassia quamash), purple coneflower (Echinacea angustifolia), chokecherry (Prunus virginiana), red raspberry (Rubus idaeus L.), huckleberries (Vaccinium spp.), kinnick-kinnick (Arcostaphylos uva-ursi), wood’s rose (Rosa woodsii), wild strawberry (Fragaria virginiana), arrowleaf balsamroot (Balsamorhiza sagittata), and fireweed (Epilobium angustifolium), in addition to deer, elk, and other game. Climate change may reduce availability of these foods and plants, as well as shift gathering sites to locations not under tribal control (Voggesser et al. 2013). For example, Holden et al. (2012) showed huckleberry and serviceberry (Amelanchier alnifolia) productivity is sensitive to climate variation. Forest changes may also impact sacred sites. For example, some sacred sites are named after the long-standing vegetation communities grown in that area; yet many of the species may no longer be present under future climates. Native people have harvested camas (Camassia quamash) for millennia as a food source in Montana. Here, native youth dig for camas roots as part of the 2011 Northwest Montana Native Youth Conservation Corps. Courtesy of US Fish and Wildlife Service. Timber production and wood industry.—Potential climate change-induced impacts to commercial forestry have been reviewed in several places (e.g., Kirilenko and Sedjo 2007; Vose et al. 2016; Keane et al. forthcoming), as has the status of Montana’s forest products industry (e.g., McIver et al. 2013). In summary, potential increases in forest productivity from climate shifts (Lin et al. 2010; NPS 2010) could result in increased timber production in Montana (Garcia-Gonzalo et al. 2007). However, decreased vigor and increased mortality might offset gains in productivity from climate alone (Kirilenko and Sedjo 2007; Vose et al. 2016). Increased diseases, insects, and fires could also reduce quality of timber, thereby reducing the value (Spittlehouse and Stewart 2004; Kirilenko and Sedjo 2007; Gillette et al. 2014). |
|
|
RECOMMENDED FURTHER READING
Keane RE, Mahalovich MF, Bollenbacher B, Manning M, Loehman R, Jain T, Holsinger LM, Larson A, Grahman R, Webster M. [forthcoming]. Forest vegetation [chapter]. Northern Rockies vulnerability assessment and adaptation plan (NRAP). Fort Collins CO: USDA Forest Service, Rocky Mountain Research Station. Report # RMRS-GTR-xxx.
Vose JM, Clark JS, Luce CH, Patel-Weynand T, editors. 2016. Effects of drought on forests and rangelands in the United States: a comprehensive science synthesis. Washington DC: USDA, Forest Service, Washington Office. General Technical Report WO-93b. 289 p.
Vose JM, Peterson DL, Patel-Weynand T, editors. 2012. Effects of climatic variability and change on forest ecosystems: a comprehensive science synthesis for the US. Portland OR: USDA Forest Service, Pacific Northwest Research Station. General Technical Report PNW-GTR-870. 282 p.
On forest mortality and climate change:
van Mantgem PJ, Stephenson NL, Byrne JC, Daniels LD, Franklin JF, Fulé PZ, Harmon ME, Larson AJ, Smith JM, Taylor AH, Veblen TT. 2009. Widespread increase of tree mortality rates in the western United States. Science 323:521–4.
On forest fire and climate change:
Abatzoglou JT, Williams AP. 2016. Impact of anthropogenic climate change on wildfire across western US forests. Proceedings of the National Academy of Sciences 113:11770–5.
On bark beetles and climate change:
Buotte PC, Hicke JA, Preisler HK, Abatzoglou JT, Raffa KF, Logan JA. 2016. Climate influences on whitebark pine mortality from mountain pine beetle in the Greater Yellowstone Ecosystem. Ecological Applications 26:2505–22.
On feedbacks, forests, and climate change:
Bonan GB. 2008. Forests and climate change: forcings, feedbacks, and the climate benefits of forests. Science 320:1444–9.
On forest management for climate change adaptation:
Colloff MJ, Doherty MD, Lavorel S, Dunlop M, Wise RM, Prober SM. 2016. Adaptation services and pathways for the management of temperate montane forests under transformational climate change. Climatic Change 138:267–82.
LITERATURE CITED
Abatzoglou JT, Kolden CA. 2013. Relationships between climate and macroscale area burned in the western United States. International Journal of Wildland Fire 22:1003–20.
Abatzoglou JT, Williams AP. 2016. Impact of anthropogenic climate change on wildfire across western US forests. Proceedings of the National Academy of Sciences 113:11770–5.
Aitken SN, Whitlock MC. 2013. Assisted gene flow to facilitate local adaptation to climate change. Annual Review of Ecology, Evolution, and Systematics 44:367–88.
Alfaro RI, Fady B, Vendramin GG, Dawson IK, Fleming RA, Sáenz-Romero C, Lindig-Cisneros RA, Murdock T, Vinceti B, Navarro CM, Skrøppa T, Baldinelli G, El-Kassaby YA, Loo J. 2014. The role of forest genetic resources in responding to biotic and abiotic factors in the context of anthropogenic climate change. Forest Ecology and Management 333:76–87.
Allen CD, Macalady AK, Chenchouni H, Bachelet D, McDowell N, Vennetier M, Kitzberger T, Rigling A, Breshears DD, Hogg EH, and 10 more. 2010. A global overview of drought and heat-induced tree mortality reveals emerging climate change risks for forests. Forest Ecology and Management 259:660–84.
Allen CD, Savage M, Falk DA, Suckling KF, Swetnam TW, Schulke T, Stacey PB, Morgan P, Hoffman M, Klingel JT. 2002. Ecological restoration of southwestern ponderosa pine ecosystems: a broad perspective. Ecological Applications 12:1418–33.
Baker WL. 2009. Fire ecology in Rocky Mountain landscapes. 1st ed. Washington DC: Island Press. 628 p.
Baldocchi D. 2008. “Breathing” of the terrestrial biosphere: lessons learned from a global network of carbon dioxide flux measurement systems. Australian Journal of Botany 56:1–26.
Bellassen V, Luyssaert S. 2014. Carbon sequestration: managing forests in uncertain times. Nature 506:153–5.
Bentz BJ, Duncan JP, Powell JA. 2016. Elevational shifts in thermal suitability for mountain pine beetle population growth in a changing climate. Forestry 89(3):271-83.
Bentz BJ, Jönsson AM. 2015. Modeling bark beetle responses to climate change [chapter]. In: Vega FE, Hofstetter RW, editors. Bark Beetles. San Diego: Academic Press. p 533–53.
Bentz BJ, Mullins DE. 1999. Ecology of mountain pine beetle (Coleoptera: Scolytidae) cold hardening in the Intermountain West. Environmental Entomology. 28:577–87.
Berner LT, Law BE, Meddens AJH, Hicke JA. [forthcoming]. Forest carbon stocks, productivity, and tree mortality from fire and bark beetles during a dry decade in the western United States (2003-2012).
Bhuta AAR, Kennedy LM, Pederson N. 2009. Climate-radial growth relationships of northern latitudinal range margin longleaf pine (Pinus palustris P. Mill.) in the Atlantic coastal plain of southeastern Virginia. Tree-Ring Research 65:105–15.
Birdsey RA. 1992. Carbon storage and accumulation in United States forest ecosystems. Washington DC: USDA Forest Service. General Technical Report WO-59. 55 p.
Bonan GB. 2008. Forests and climate change: forcings, feedbacks, and the climate benefits of forests. Science 320:1444–9.
Breshears DD, Cobb NS, Rich PM, Price KP, Allen CD, Balice RG, Romme WH, Kastens JH, Floyd ML, Belnap J, Anderson JJ, Myers OB, Meyer CW. 2005. Regional vegetation die-off in response to global-change-type drought. Proceedings of the National Academy of Sciences 102:15144–8.
Brookshire ENJ, Gerber S, Webster JR, Vose JM, Swank WT. 2011. Direct effects of temperature on forest nitrogen cycling revealed through analysis of long-term watershed records. Global Change Biology 17:297–308.
Brunelle A, Whitlock C, Bartlein PJ, Kipfmuller K. 2005. Postglacial fire, climate, and vegetation history along an environmental gradient in the northern Rocky Mountains. Quaternary Science Reviews 24:2281-300.
Buma B. 2015. Disturbance interactions: characterization, prediction, and the potential for cascading effects. Ecosphere 6:1–15.
Buotte PC, Hicke JA, Preisler HK, Abatzoglou JT, Raffa KF, Logan JA. 2016. Climate influences on whitebark pine mortality from mountain pine beetle in the Greater Yellowstone Ecosystem. Ecological Applications 26:2505–22.
Carroll AL, Régnière J, Logan JA, Taylor SW, Bentz BJ, Powell JA. 2006. Impacts of climate change on range expansion by the mountain pine beetle. Canadian Forest Service Publications Catalog ID: 26601. 27 p. Available online https://cfs.nrcan.gc.ca/publications?id=26601. Accessed 2016 May 23.
Cayan DR, Das T, Pierce DW, Barnett TP, Tyree M, Gershunov A. 2010. Future dryness in the southwest US and the hydrology of the early 21st century drought. Proceedings of the National Academy of Sciences 107:21271–6.
Chakraborty S, Luck J, Freeman A, Norton RM, Garrett KA, Percy KE, Hopkin AA, Davis CN, Karnosky DF. 2008. Impacts of global change on diseases of agricultural crops and forest types. CAB Reviews: Perspectives in agriculture, veterinary science, nutrition, and natural resources 3(54):1-15.
Charney ND, Babst F, Poulter B, Record S, Trouet VM, Frank D, Enquist BJ, Evans MEK. 2016. Observed forest sensitivity to climate implies large changes in 21st century North American forest growth. Ecological Letters 19(9):1119-28.
Cheliak WM, Murray G, Pitel JA. 1988. Genetic effects of phenotypic selection in white spruce. Forest Ecology and Management 24:139–49.
Chmura DJ, Anderson PD, Howe GT, Harrington CA, Halofsky JE, Peterson DL, Shaw DC, St Clair BJ. 2011. Forest responses to climate change in the northwestern United States: ecophysiological foundations for adaptive management. Forest Ecology and Management 261:1121–42.
Clark JS, Iverson L, Woodall CW, Allen CD, Bell DM, Bragg DC, D’Amato AW, Davis FW, Hersh MH, Ibanez I, and 7 more. 2016. The impacts of increasing drought on forest dynamics, structure, and biodiversity in the United States. Global Change Biology 22:2329-52.
Compant S, van der Heijden MGA, Sessitsch A. 2010. Climate change effects on beneficial plant-microorganism interactions. FEMS Microbiology Ecology 73:197–214.
Coops NC, Waring RH. 2011. Estimating the vulnerability of fifteen tree species under changing climate in northwest North America. Ecological Modelling 222(13):2119-29.
Creeden EP, Hicke JA, Buotte PC. 2014. Climate, weather, and recent mountain pine beetle outbreaks in the western United States. Forest Ecology and Management 312:239–51.
Crimmins SM, Dobrowski SZ, Greenberg JA, Abatzoglou JT, Mynsberge AR. 2011. Changes in climatic water balance drive downhill shifts in plant species’ optimum elevations. Science 331:324–7.
Dale VH, Joyce LA, McNulty S, Neilson RP, Ayres MP, Flannigan MD, Hanson PJ, Irland LC, Lugo AE, Peterson CJ, Simberloff D, Swanson FJ, Stocks BJ, Wooton BM. 2001. Climate change and forest disturbances. BioScience 51:723–34.
Davidson EA, Janssens IA. 2006. Temperature sensitivity of soil carbon decomposition and feedbacks to climate change. Nature 440:165–73.
Davis MB, Shaw RG. 2001. Range shifts and adaptive responses to quaternary climate change. Science 292:673–9.
Davis MB, Shaw RG, Etterson JR. 2005. Evolutionary responses to changing climate. Ecology 86:1704–14.
Dennison PE, Brewer SC, Arnold JD, Moritz MA. 2014. Large wildfire trends in the western United States, 1984–2011. Geophysical Research Letters 41:2928-33.
Desprez-Loustau M-L, Marçais B, Nageleisen L-M, Piou D, Vannini A. 2006. Interactive effects of drought and pathogens in forest trees. Annals of Forest Science 63:16.
Diffenbaugh NS, Field CB. 2013. Changes in ecologically critical terrestrial climate conditions. Science 341:486–92.
Dobrowski SZ, Abatzoglou J, Swanson AK, Greenberg JA, Mynsberge AR, Holden ZA, Schwartz MK. 2013. The climate velocity of the contiguous United States during the 20th century. Global Change Biology 19:241–51.
Dobrowski SZ, Swanson AK, Abatzoglou JT, Holden ZA, Safford HD, Schwartz MK, Gavin DG. 2015. Forest structure and species traits mediate projected recruitment declines in western US tree species. Global Ecology and Biogeography 24:917–27.
D’Orangeville L, Duchesne L, Houle D, Kneeshaw D, Côté B, Pederson N. 2016. Northeastern North America as a potential refugium for boreal forests in a warming climate. Science 352:1452–5.
Ehleringer JR, Cerling TE. 1995. Atmospheric CO2 and the ratio of intercellular to ambient CO2 concentrations in plants. Tree Physiology 15:105–11.
Erickson V, Aubry C, Berrang P, Blush T, Bower A, Crane B, DeSpain T, Gwaze D, Hamlin J, Horning M, Johnson R, Mahalovich M, Maldonado M, Sniezko R, St. Clair B. 2012. Genetic resource management and climate change: genetic options for adapting national forests to climate change. Washington DC: USDA Forest Service, Forest Management. Report # stelprdb1077125. 24 p.
[FAO] Food and Agriculture Organization of the United Nations. 2014. The state of the world’s forest genetic resources. 304 p. Rome Italy: Commission on Genetic Resources for Food and Agriculture. Available online http://www.fao.org/3/a-i3825e.pdf. Accessed 2017 May 10.
Finzi AC, Moore DJP, DeLucia EH, Lichter J, Hofmockel KS, Jackson RB, Kim H-S, Matamala R, McCarthy HR, Oren R, Pippen JS, Schlessinger WH. 2006. Progressive nitrogen limitation of ecosystem processes under elevated CO2 in a warm-temperate forest. Ecology 87:15–25.
Flannigan MD, Harrington JB. 1988. A study of the relation of meteorological variables to monthly provincial area burned by wildfire in Canada (1953–80). Journal of Applied Meteorology 27:441–52.
Flower A, Gavin DG, Heyerdahl EK, Parsons RA, Cohn GM. 2014. Drought-triggered western spruce budworm outbreaks in the interior Pacific Northwest: a multi-century dendrochronological record. Forest Ecology and Management 324:16–27.
Ford KR, Breckheimer IK, Franklin JF, Freund JA, Kroiss SJ, Larson AJ, Theobald EJ, HilleRisLambers J. 2016. Competition alters tree growth responses to climate at individual and stand scales. Canadian Journal of Forest Research 47: 53-62.
Franks PJ, Adams MA, Amthor JS, Barbour MM, Berry JA, Ellsworth DS, Farquhar GD, Ghannoum O, Lloyd J, McDowell N, Norby RJ, Tissue DT, von Caemmerer S. 2013. Sensitivity of plants to changing atmospheric CO2 concentration: from the geological past to the next century. New Phytologist 197:1077–94.
Galiano L, Martínez-Vilalta J, Eugenio M, Granzow-de la Cerda Í, Lloret F. 2013. Seedling emergence and growth of Quercus spp. following severe drought effects on a Pinus sylvestris canopy. Journal of Vegetation Science 24:580–8.
Ganey JL, Vojta SC. 2011. Tree mortality in drought-stressed mixed-conifer and ponderosa pine forests, Arizona, USA. Forest Ecology and Management 261:162–8.
Garcia-Gonzalo J, Peltola H, Briceño-Elizondo E, Kellomäki S. 2007. Effects of climate change and management on timber yield in boreal forests, with economic implications: a case study. Ecological Modelling 209:220–34.
Garten CT, Iversen CM, Norby RJ. 2011. Litterfall 15N abundance indicates declining soil nitrogen availability in a free-air CO2 enrichment experiment. Ecology 92:133–9.
Gedalof Z, Berg AA. 2010. Tree ring evidence for limited direct CO2 fertilization of forests over the 20th century. Global Biogeochemical Cycles 24:GB3027.
Gillette NE, Wood DL, Hines SJ, Runyon JB, Negron JF. 2014. The once and future forest: consequences of mountain pine beetle treatment decisions. Forest Science 60:527–38.
Gottfried M, Pauli H, Futschik A, Akhalkatsi M, Barančok P, Benito Alonso JL, Coldea G, Dick J, Erschbamer B, Fernández Calzado MR, and 22 more. 2012. Continent-wide response of mountain vegetation to climate change. Nature Climate Change 2:111–5.
Guardiola-Claramonte M, Troch PA, Breshears DD, Huxman TE, Switanek MB, Durcik M, Cobb NS. 2011. Decreased streamflow in semi-arid basins following drought-induced tree die-off: a counter-intuitive and indirect climate impact on hydrology. Journal of Hydrology 406:225–33.
Heyerdahl EK, Brubaker LB, Agee JK. 2002. Annual and decadal climate forcing of historical fire regimes in the interior Pacific Northwest, USA. The Holocene 12:597–604.
Heyerdahl EK, Morgan P, Riser JP. 2008. Multi-season climate synchronized historical fires in dry forests (1650–1900), Northern Rockies, USA. Ecology 89:705–16.
Hicke JA, Logan JA, Powell J, Ojima DS. 2006. Changing temperatures influence suitability for modeled mountain pine beetle (Dendroctonus ponderosae) outbreaks in the western United States. Journal of Geophysical Research-Biogeosciences 111:G02019.
Hoff V. [forthcoming]. A Montana fire atlas. Data collected by and available from the National Center for Landscape Fire Analysis, University of Montana. Funding provided by the Montana Land Information Advisory Council.
Holden ZA, Jolly WM. 2011. Modeling topographic influences on fuel moisture and fire danger in complex terrain to improve wildland fire management decision support. Forest Ecology and Management 262:2133-41.
Holden ZA, Kasworm WF, Servheen C, Hahn B, Dobrowski S. 2012. Sensitivity of berry productivity to climatic variation in the Cabinet–Yaak grizzly bear recovery zone, northwest United States, 1989–2010. Wildlife Society Bulletin 36:226–31.
Ibáñez I, Clark JS, LaDeau S, Lambers JHR. 2007. Exploiting temporal variability to understand tree recruitment response to climate change. Ecological Monographs 77:163–77.
Ibáñez I, McCarthy-Neumann S. 2014. Integrated assessment of the direct and indirect effects of resource gradients on tree species recruitment. Ecology 95:364–75.
Ingerson A. 2007. US forest carbon and climate change: controversies and win-win policy approaches. Washington DC: The Wilderness Society. 28 p. Available online http://www.nrcm.org/wp-content/uploads/2013/10/TWS_US-Forest-Carbon-and-.... Accessed 2017 May 10.
Jolly WM, Cochrane MA, Freeborn PH, Holden ZA, Brown TJ, Williamson GJ, Bowman DMJS. 2015. Climate-induced variations in global wildfire danger from 1979 to 2013. Nature Communications 6:7537.
Joyce LA, Birdsey RA. 1995. Productivity of America’s forests and climate change. Fort Collins CO: USDA Forest Service, Rocky Mountain Research Station. Report # RM-GTR-271. 73 p.
Keane RE, Mahalovich MF, Bollenbacher B, Manning M, Loehman R, Jain T, Holsinger LM, Larson A, Grahman R, Webster M. [forthcoming]. Forest vegetation [chapter]. Northern Rockies vulnerability assessment and adaptation plan (NRAP). Fort Collins CO: USDA Forest Service, Rocky Mountain Research Station. Report # RMRS-GTR-xxx.
Kirilenko AP, Sedjo RA. 2007. Climate change impacts on forestry. Proceedings of the National Academy of Sciences 104:19697–702.
Kolb PF, Robberecht R. 1996. High temperature and drought stress effects on survival of Pinus ponderosa seedlings. Tree Physiology 16:665–72.
Krist FJ, Ellenwood JR, Woods ME, McMahan AJ, Cowardin JP, Ryerson DE, Sapio FJ, Zweifler MO, Romero SA. 2014. 2013-2027 national insect and disease forest risk assessment. Fort Collins CO: USDA Forest Service. Report # FHTET-14-01. 209 p.
Kurz WA, Dymond CC, Stinson G, Rampley GJ, Neilson ET, Carroll AL, Ebata T, Safranyik L. 2008. Mountain pine beetle and forest carbon feedback to climate change. Nature 452:987–90.
Ladeau SL, Clark JS. 2006. Pollen production by Pinus taeda growing in elevated atmospheric CO2. Functional Ecology 20:541–7.
Landfire. 2012. Existing vegetation cover data v1.3.0 [website]. Available online https://www.landfire.gov/index.php#. Accessed 2017 May 10.
League K, Veblen T. 2006. Climatic variability and episodic Pinus ponderosa establishment along the forest-grassland ecotones of Colorado. Forest Ecology and Management 228:98–107.
Ledig FT, Kitzmiller JH. 1992. Genetic strategies for reforestation in the face of global climate change. Forest Ecology and Management 50:153–69.
Lin D, Xia J, Wan S. 2010. Climate warming and biomass accumulation of terrestrial plants: a meta-analysis. New Phytologist 188:187–98.
Littell JS, Gwozdz RB. 2011. Climatic water balance and regional fire years in the Pacific Northwest, USA: linking regional climate and fire at landscape scales [chapter]. In: McKenzie D, Miller C, Falk DA, editors. The landscape ecology of fire. Netherlands: Springer. p. 117–39.
Littell JS, McKenzie D, Peterson DL, Westerling AL. 2009. Climate and wildfire area burned in western US ecoprovinces, 1916–2003. Ecological Applications 19:1003–21.
Littell JS, Peterson DL, Tjoelker M. 2008. Douglas-fir growth in mountain ecosystems: water limits tree growth from stand to region. Ecological Monographs 78:349–68.
Loehman RA, Reinhardt E, Riley KL. 2014. Wildland fire emissions, carbon, and climate: seeing the forest and the trees—a cross-scale assessment of wildfire and carbon dynamics in fire-prone, forested ecosystems. Forest Ecology and Management 317:9–19.
Lorio PLJ. 1993. Environmental stress and whole-tree physiology. In: Showalter T, Filip G, editors. Beetle-pathogen interactions in conifer forests. London: Academic Press. p 81–101.
Luo Y, Chen HYH. 2013. Observations from old forests underestimate climate change effects on tree mortality. Nature Communications 4:1655. doi:10.1038/ncomms2681.
Marlon JR, Bartlein PJ, Carcaillet C, Gavin DG, Harrison SP, Higuera PE, Joos F, Power MJ, Prentice IC. 2008. Climate and human influences on global biomass burning over the past two millennia. Nature Geoscience 1:697–702.
Marlon JR, Bartlein PJ, Gavin DG, Long CJ, Anderson RS, Briles CE, Brown KJ, Colombaroli D, Hallett DJ, Power MJ, Scharf EA, Walsh MK. 2012. Long-term perspective on wildfires in the western USA. Proceedings of the National Academy of Sciences 109:E535–E543.
McIver CP, Sorenson CB, Keegan CE, Morgan TA, Menlove J. 2013. Montana’s forest products industry and timber harvest, 2009. Fort Collins CO: USDA Forest Service, Rocky Mountain Research Station. Resource Bulletin RMRS-RB-16. 49 p.
McKenney D, Pedlar J, O’Neill G. 2009. Climate change and forest seed zones: past trends, future prospects and challenges to ponder. The Forestry Chronicle 85:258–66.
McKenzie D, Littell JS. 2017. Climate change and the eco-hydrology of fire: will area burned increase in a warming western US? Ecological Applications 27:26-36.
McKinley DC, Ryan MG, Birdsey RA, Giardina CP, Harmon ME, Heath LS, Houghton RA, Jackson RB, Morrison JF, Murray BC, Pataki DE, Skog KE. 2011. A synthesis of current knowledge on forests and carbon storage in the United States. Ecological Applications 21:1902–24.
McLachlan JS, Hellmann JJ, Schwartz MW. 2007. A framework for debate of assisted migration in an era of climate change. Conservation Biology 21:297–302.
McMahon SM, Parker GG, Miller DR. 2010. Evidence for a recent increase in forest growth. Proceedings of the National Academy of Sciences 107:3611–5.
Melillo JM, Butler S, Johnson J, Mohan J, Steudler P, Lux H, Burrows E, Bowles F, Smith R, Scott L, Varioa C, Hill T, Burton A, Zhou Y-M, Tang T. 2011. Soil warming, carbon–nitrogen interactions, and forest carbon budgets. Proceedings of the National Academy of Sciences 108:9508–12.
Metsaranta JM, Kurz WA, Neilson ET, Stinson G. 2010. Implications of future disturbance regimes on the carbon balance of Canada’s managed forest (2010-2100). Telus 62:719-28.
[MFWP] Montana Fish, Wildlife & Parks. 2016 Sep 22. Yellowstone River fish kill fact sheet—updated Sept. 22, 2016 [website press release]. Helena MT: State of Montana; Montana Fish, Wildlife & Parks. Available online http://fwp.mt.gov/news/newsReleases/closures/waterbodies/nr_106.html. Accessed 2017 May 30.
Millar CI, Stephenson NL, Stephens SL. 2007. Climate change and forests of the future: managing in the face of uncertainty. Ecological Applications 17:2145–51.
Miller JD, Safford HD, Crimmins M, Thode AE. 2008. Quantitative evidence for increasing forest fire severity in the Sierra Nevada and southern Cascade mountains, California and Nevada, USA. Ecosystems 12:16–32.
Mitton JB, Ferrenberg SM. 2012. Mountain pine beetle develops an unprecedented summer generation in response to climate warming. The American Naturalist 179(5):E163–E171.
Monleon VJ, Lintz HE. 2015. Evidence of tree species’ range shifts in a complex landscape. PLoS ONE 10(1):e0118069.
Morgan P, Heyerdahl EK, Gibson CE. 2008. Multi-season climate synchronized forest fires throughout the 20th century, northern Rockies, USA. Ecology 89:717–28.
Moritz C, Agudo R. 2013. The future of species under climate change: resilience or decline? Science 341:504–8.
[MT DNRC] Montana Department of Natural Resources and Conservation. 2010. Montana’s state assessment of forest resources. Missoula MT: DNRC. 29 p. Available online http://dnrc.mt.gov/divisions/forestry/docs/assistance/samethodology2010.pdf. Accessed 2017 May 10.
Nicotra AB, Beever EA, Robertson AL, Hofmann GE, O’Leary J. 2015. Assessing the components of adaptive capacity to improve conservation and management efforts under global change. Conservation Biology 5:1268–78.
[NIFC] National Interagency Fire Center. [undated]. Fire and weather data [website]. Available online https://fam.nwcg.gov/fam-web/weatherfirecd/. Accessed 2017 April 4.
Norby RJ, Warren JM, Iversen CM, Medlyn BE, McMurtrie RE. 2010. CO2 enhancement of forest productivity constrained by limited nitrogen availability. Proceedings of the National Academy of Sciences 107:19368–73.
[NPS] US Department of the Interior, National Park Service. 2010. Observed and projected ecological response to climate change in the Rocky Mountains and Upper Columbia Basin : a synthesis of current scientific literature. Fort Collins CO: National Park Service. Natural Resource Report # NPS/ROMN/NRR—2010/220. 98 p.
O’Connor CD. 2013. Spatial and temporal dynamics of disturbance interactions along an ecological gradient [PhD dissertation]. Tempe AZ: University of Arizona. 204 p. Available online https://www.fs.fed.us/rm/pubs_journals/2013/rmrs_2013_oconnor_c001.pdf. Accessed 2017 May 10.
Pan Y, Birdsey RA, Fang J, Houghton R, Kauppi PE, Kurz WA, Phillips OL, Shvidenko A, Lewis SL, Canadell JG, and 8 more. 2011. A large and persistent carbon sink in the world’s forests. Science 333:988–93.
Parisien MA, Moritz MA. 2009. Environmental controls on the distribution of wildfire at multiple spatial scales. Ecological Monographs 79:127–54.
Parks SA, Holsinger LM, Miller C, Nelson CR. 2015. Wildland fire as a self-regulating mechanism: the role of previous burns and weather in limiting fire progression. Ecological Applications 25:1478–92.
Peterson DL, Millar CI, Joyce LA, Furniss MJ, Halofsky JE, Neilson RP, Morelli TL. 2011. Responding to climate change in national forests: a guidebook for developing adaptation options. Portland OR: USDA Forest Service, Pacific Northwest Research Station. Report # PNW-GTR-855. 118 p.
Petrie MD, Wildeman AM, Bradford JB, Hubbard RM, Lauenroth WK. 2016. A review of precipitation and temperature control on seedling emergence and establishment for ponderosa and lodgepole pine forest regeneration. Forest Ecology and Management 361:328–38.
Piao S, Ciais P, Friedlingstein P, Peylin P, Reichstein M, Luyssaert S, Margolis H, Fang J, Barr A, Chen A, and 6 more. 2008. Net carbon dioxide losses of northern ecosystems in response to autumn warming. Nature 451:49–52.
Power MJ, Marlon J, Ortiz N, Bartlein PJ, Harrison SP, Mayle FE, Ballouche A, Bradshaw RHW, Carcaillet C, Cordova C, and 74 more. 2007. Changes in fire regimes since the Last Glacial Maximum: an assessment based on a global synthesis and analysis of charcoal data. Climate Dynamics 30:887–907.
Power MJ, Whitlock C, Bartlein PJ. 2011. Postglacial fire, vegetation, and climate history across an elevational gradient in the northern Rocky Mountains, USA and Canada. Quaternary Science Reviews 30:2520-33.
Preisler HK, Hicke JA, Ager AA, Hayes JL. 2012. Climate and weather influences on spatial temporal patterns of mountain pine beetle populations in Washington and Oregon. Ecology 93:2421–34.
Raffa KF, Powell EN, Townsend PA. 2013. Temperature-driven range expansion of an irruptive insect heightened by weakly coevolved plant defenses. Proceedings of the National Academy of Sciences 110:2193–8.
Rapacciuolo G, Maher SP, Schneider AC, Hammond TT, Jabis MD, Walsh RE, Iknayan KJ, Walden GK, Oldfather MF, Ackerly DD, Beissinger SR. 2014. Beyond a warming fingerprint: individualistic biogeographic responses to heterogeneous climate change in California. Global Change Biology 20:2841–55.
Régnière J, Bentz B. 2007. Modeling cold tolerance in the mountain pine beetle, Dendroctonus ponderosae. Journal of Insect Physiology 53:559–72.
Régnière J, St-Amant R, Duval P. 2010. Predicting insect distributions under climate change from physiological responses: spruce budworm as an example. Biological Invasions 14:1571–86.
Richardson AD, Keenan TF, Migliavacca M, Ryu Y, Sonnentag O, Toomey M. 2013. Climate change, phenology, and phenological control of vegetation feedbacks to the climate system. Agricultural and Forest Meteorology169:156–73.
Rocca ME, Brown PM, MacDonald LH, Carrico CM. 2014. Climate change impacts on fire regimes and key ecosystem services in Rocky Mountain forests. Forest Ecology and Management 327:290–305.
Ryan MG, Gower ST, Hubbard RM, Waring RH, Gholz HL, Cropper Jr WP, Running SW. 1995. Woody tissue maintenance respiration of four conifers in contrasting climates. Oecologia 101:133–40.
Salzer MW, Hughes MK, Bunn AG, Kipfmueller KF. 2009. Recent unprecedented tree-ring growth in bristlecone pine at the highest elevations and possible causes. Proceedings of the National Academy of Sciences 106:20348–53.
Schaberg PG, DeHayes DH, Hawley GJ, Nijensohn SE. 2008. Anthropogenic alterations of genetic diversity within tree populations: implications for forest ecosystem resilience. Forest Ecology and Management 256:855–62.
Schaedel MS, Larson AJ, Affleck DL, Belote RT, Goodburn JM, Page-Dumroese DS. 2017. Early forest thinning changes aboveground carbon distribution among pools, but not total amount. Forest Ecology and Management 389:187–98.
Schoennagel T, Veblen TT, Romme WH. 2004. The interaction of fire, fuels, and climate across Rocky Mountain forests. BioScience 54:661–76.
Schueler S, Kapeller S, Konrad H, Geburek T, Mengl M, Bozzano M, Koskela J, Lefèvre F, Hubert J, Kraigher H, Roman Longauer R, Olrik DC. 2012. Adaptive genetic diversity of trees for forest conservation in a future climate: a case study on Norway spruce in Austria. Biodiversity and Conservation 22:1151–66.
Seager R, Hooks A, Williams AP, Cook B, Nakamura J, Henderson N. 2015. Climatology, variability, and trends in the US vapor pressure deficit, an important fire-related reteorological quantity. Journal of Applied Meteorology and Climatology 54:1121–41.
Shafer SL, Bartlein PJ, Gray EM, Pelltier RT. 2015. Projected future vegetation changes for the northwest United States and southwest Canada at a fine spatial resolution using a dynamic global vegetation model. PLoS ONE 10(10):e0138759.
Sharples JJ. 2009. An overview of mountain meteorological effects relevant to fire behaviour and bushfire risk. International Journal of Wildland Fire 18:737–54.
Smith CM, Wilson B, Rasheed S, Walker RC, Carolin T, Shepherd B. 2008. Whitebark pine and white pine blister rust in the Rocky Mountains of Canada and northern Montana. Canadian Journalof Forest Research 38:982–95.
Solomon S, Plattner G-K, Knutti R, Friedlingstein P. 2009. Irreversible climate change due to carbon dioxide emissions. Proceedings of the National Academy of Sciences 106:1704–9.
Spittlehouse DL, Stewart RB. 2004. Adaptation to climate change in forest management. Journal of Ecosystems and Management 4(1). Available online http://jem.forrex.org/index.php/jem/article/view/254/173. Accessed 2017 May 10.
Sturrock RN, Frankel SJ, Brown AV, Hennon PE, Kliejunas JT, Lewis KJ, Worrall JJ, Woods AJ. 2011. Climate change and forest diseases. Plant Pathology 60:133–49.
Swanston CW, Janowiak MK, Brandt LA, Butler PR, Handler SD, Shannon PD, Derby Lewis A, Hall K, Fahey RT, Scott L, et al. 2016. Forest adaptation resources: climate change tools and approaches for land managers. 2nd ed. Newtown Square PA: US Department of Agriculture, US Forest Service, Northern Research Station. Report # GTR-NRS-87-2.
Swetnam TW, Betancourt JL. 2010. Mesoscale disturbance and ecological response to decadal climatic variability in the American Southwest [chapter]. In: Stoffel M, Bollschweiler M, Butler DR, Luckman BH, editors. Tree rings and natural hazards: a state-of-art. Netherlands: Springer. p 329–59.
Trenberth KE, Dai A, van der Schrier G, Jones PD, Barichivich J, Briffa KR, Sheffield J. 2014. Global warming and changes in drought. Nature Climate Change 4:17–22.
[USFS] US Department of Agriculture, Forest Service. 2015. Baseline estimates of carbon stocks in forests and harvested wood products for National forest system units: Northern Region [whitepaper]. 43 p. Available online https://www.fs.fed.us/climatechange/documents/NorthernRegionCarbonAssess.... Accessed 2017 May 10.
[USFS] US Department of Agriculture, Forest Service. 2016. United States Forest Service aerial detection survey (ADS) data for years 2000-2015 [website]. Available online https://www.fs.usda.gov/detailfull/r1/landmanagement/gis/?cid=stelprdb54.... Access 2017 May 10.
van Mantgem PJ, Nesmith JCB, Keifer M, Knapp EE, Flint A, Flint L. 2013. Climatic stress increases forest fire severity across the western United States. Ecological Letters 16:1151–6.
van Mantgem PJ, Stephenson NL, Byrne JC, Daniels LD, Franklin JF, Fulé PZ, Harmon ME, Larson AJ, Smith JM, Taylor AH, Veblen TT. 2009. Widespread increase of tree mortality rates in the western United States. Science 323:521–4.
Voggesser G, Lynn K, Daigle J, Lake FK, Ranco D. 2013. Cultural impacts to tribes from climate change influences on forests. Climatic Change 120:615–26.
Vose JM, Clark JS, Luce CH, Patel-Weynand T, editors. 2016. Effects of drought on forests and rangelands in the United States: a comprehensive science synthesis. Washington DC: USDA, Forest Service, Washington Office. General Technical Report WO-93b. 289 p.
Vose JM, Peterson DL, Patel-Weynand T, editors. 2012. Effects of climatic variability and change on forest ecosystems: a comprehensive science synthesis for the US. Portland OR: USDA Forest Service, Pacific Northwest Research Station. General Technical Report PNW-GTR-870. 282 p.
Waring RH, Running SW. 2007. Forest ecosystems: analysis at multiple scales. 3rd ed. Amsterdam: Elsevier, Academic Press. 440 p.
Wear DN, Coulston JW. 2015. From sink to source: regional variation in US forest carbon futures. Scientific Reports 5:16518. doi:10.1038/srep16518.
Weed AS, Ayres MP, Hicke JA. 2013. Consequences of climate change for biotic disturbances in North American forests. Ecological Monographs 83:441–70.
Westerling AL, Hidalgo HG, Cayan DR, Swetnam TW. 2006. Warming and earlier spring increase western US forest wildfire activity. Science 313:940–3.
Westerling AL, Turner MG, Smithwick EAH, Romme WH, Ryan MG. 2011. Continued warming could transform Greater Yellowstone fire regimes by mid-21st century. Proceedings of the National Academy of Sciences 108:13165–70.
Westfall RD, Millar CI. 2004. Genetic consequences of forest population dynamics influenced by historic climatic variability in the western USA. Forest Ecology and Management 197:159–70.
Whitlock C. 1993. Postglacial vegetation and climate of Grand Teton and southern Yellowstone National Parks. Ecological Monographs 63:173-98.
Williams AP, Allen CD, Macalady AK, Griffin D, Woodhouse CA, Meko DM, Swetnam TW, Rauscher SA, Seager R, Grissino-Mayer HD, and 5 more. 2013. Temperature as a potent driver of regional forest drought stress and tree mortality. Nature Climate Change 3:292–7.
Worrall JJ, Rehfeldt GE, Hamann A, Hogg EH, Marchetti SB, Michaelian M, Gray LK. 2013. Recent declines of Populus tremuloides in North America linked to climate. Forest Ecology and Management 299:35–51.
Wright M. 2016 August 19. Yellowstone River closed following unprecedented fish kill. Bozeman Daily Chronicle. Environment section.
Zhang YG, Pagani M, Liu Z, Bohaty SM, DeConto R. 2013. A 40-million-year history of atmospheric CO2. Philosophical Transactions of the Royal Society A 371:20130096.
Zhu K, Woodall CW, Clark JS. 2012. Failure to migrate: lack of tree range expansion in response to climate change. Global Change Biology 18:1042–52.
22 This report focuses on climate change impacts, not mitigation. Still it is important to recognize that forests are a key carbon sink, and have the potential to reduce atmospheric CO2 through carbon sequestration (i.e., the removal of carbon from the atmosphere to be stored in an alternative form) (Ingerson 2007; Pan et al. 2011). Pan et al. (2011) estimated that forests offset 13% of carbon emissions in North America. Birdsey (1992) estimated Rocky Mountain forests make up about 15% of total US forest carbon storage.
23 New research indicates that there is not a trade-off between managing for productivity and carbon storage; stands managed with early (prior to onset of canopy closure and intense competition), pre-commercial thinning had lower densities, larger trees, greater structural complexity, and stored as much aboveground carbon as un-thinned stands (Schaedel et al. 2017).